Open Access
ARTICLE
Effects of Tsukamurella tyrosinosolvens P9 on growth, physiology and antioxdant enzyme of peanut under drought stress and after re-watering
College of Life Sciences, Key Laboratory of Plant Resource Conservation and Germplasm Innovation in Mountainous Region (Ministry of Education), Collaborative Innovation Center for Mountain Ecology & Agro-Bioengineering (CICMEAB), Institute of Agro-Bioengineering, Guizhou University, Guiyang, 550025, China
* Corresponding Author: Lizhen Han,
(This article belongs to the Special Issue: Physiology and Molecular Biology of Plant Stress Tolerance)
BIOCELL 2023, 47(6), 1417-1430. https://doi.org/10.32604/biocell.2023.027485
Received 01 November 2022; Accepted 31 January 2023; Issue published 19 May 2023
Abstract
Background: The plant-growth-promoting rhizobacterium Tsukamurella tyrosinosolvens is a rare strain of actinomycete, in order to recognize and expand the ecological functions of rare actinomycetes. Methods: In this experiment, we studied the effect of Tsukamurella tyrosinosolvens P9 on the drought resistance of peanut by inoculating peanut seedlings in pots and measuring the growth and physiological indicators of peanut under drought stress and re-watering conditions. Results: The results showed that during drought stress, the relative water content of the soil and leaves, chlorophyll content, and stomatal length, width, and aperture were significantly decreased while the levels of malondialdehyde (MDA), H2O2 and stomatal density were significantly increased. Peanut growth was also inhibited. However, inoculation with the P9 strain significantly promoted the growth of peanut under drought stress as plant height, fresh weight, root length and root weight were significantly higher compared with the uninoculated drought stress group. In addition, in P9-inoculated plants, the water and chlorophyll contents were significantly higher and the activities of the antioxidant enzymes CAT and SOD were significantly increased (except during the six days of drought treatment). While the stomatal length, width, and aperture were improved, the levels of MDA and H2O2 were significantly decreased. NBT staining showed that inoculation with P9 reduced O2− accumulation under stress. After re-watering, the physiological indexes of inoculated plants recovered more quickly and grew better. Conclusions: The results showed that T. tyrosinosolvens P9 enhanced drought resistance and improves peanut growth by increasing leaf water content, increasing photosynthesis, regulating stomatal closure, and improving antioxidant enzyme activity.Keywords
Peanut (Arachis hypogeae L.) is a valuable food and oil crop, which is widely cultivated due to its high oil content and adaptability (Mingrou et al., 2022). By 2019, the planting area of peanut in China was 4.51 × 106 hectares, accounting for about 50% of oil crops (Wang et al., 2022a, 2022b). Peanut mainly grows in arid and semi-arid areas; therefore, drought is an important environmental factor limiting its productivity, with annual production losses of up to 50% (Ding et al., 2022; Li et al., 2022a). Under drought stress, plants produce many reactive oxygen species (ROS), such as superoxide anion free radicals (O2·−), hydrogen peroxide (H2O2), hydroxyl radicals (HO.), and singlet oxygen (1O2), which can react with proteins, lipids, and deoxyribonucleic acids (reaction (1)) (Collin, 2019). These interactions cause physiological disorders that inhibit plant
growth, such as electrolyte leakage and lipid peroxidation, and may even lead to plant death in severe drought conditions (Kaushal and Wani, 2016). Plant growth-promoting rhizobacteria (PGPR) have attracted attention here because they can improve plant drought tolerance; thus, screening and applying PGPRs may help improve dryland production.
PGPRs are a group of microorganisms that colonize the rhizospheres of plants, promoting plant growth and enhancing stress tolerance (Khan and Singh, 2021). PGPRs can enhance plant nutrient absorption, induce osmosis and cause antioxidant accumulation through the production of plant hormones, 1-aminocyclopropane-1-carboxylate (ACC) deaminase, and volatile compounds. They can cause changes in root morphology to promote plant hormone balance and restore plant growth and development under drought conditions (Vurukonda et al., 2016; Kour et al., 2019). For example, Paenibacillus sp., Azospirillum sp., Rhizobium sp., Bacillus sp., Azotobacter sp., Klebsiella sp., Pseudomonas sp., and Serratia sp. activated the antioxidant defense system and reduce oxidative damage, while promoting plant growth under stress (Abdelaal et al., 2021). Enterobacter sp. and Chryseobacterium sp. could reduce the negative effect of ethylene on the growth and development of mung bean under stress by producing ACC deaminase to degrade the ethylene precursor ACC (Tittabutr et al., 2013). Siderophores secreted by Bacillus sp. could improve the iron uptake of potato, which improved photosynthetic performance and tolerance to abiotic stress (Gururani et al., 2013). In addition, Bacillus, Pseudomonas, Enterobacter, and Moraxella species stimulated cell growth and differentiation by synthesizing indoleacetic acid (IAA) to promote the development of lateral roots, branches, and tillers in wheat, which improved stress resistance and yield (Raheem et al., 2018). Inoculation with PGPRs is helpful for improving plant drought resistance. However, few studies on PGPRs focus on actinomycetes (Bouskill et al., 2013) that led us to explore the same.
The rare actinomycete Tsukamurella tyrosinosolvens P9 was isolated in our laboratory from the soil surrounding the rhizospheres of tea plants. We had reported for the first time that the strain possesses multiple growth-promoting properties, such as phosphorus solubilization and production of IAA, siderophores, and ACC deaminase. Moreover, it could significantly promote the growth of peanut seedlings, and alleviate salt stress (Zhang et al., 2021; Xu et al., 2022). However, whether P9 influences the growth of peanut under drought stress is unknown. In this study, we dynamically traced the effects of P9 inoculation on the growth and drought resistance of peanut seedlings under drought stress and after re-watering to analyze and elucidate the mechanism by which P9 improves the drought resistance of peanut. This lays a foundation for understanding and expanding the ecological function of rare actinomycetes and enhancing peanut drought resistance.
The Tsukamurella tyrosinosolvens P9 strain was isolated in our laboratory and stored in the China Center for Type Culture Collection (Strain preservation number: CCTCC AA 2020052).
The peanut variety is the Silihong peanut, which is an early maturing local variety with a dark red seed coat.
The experimental soil was yellow soil and was collected in Yangniu Village (E106°39′11′′, N26°26′57′′), Guizhou Province, China. Subsequent to sieving and sterilizing three times at 121°C for one hour, it was used in pot experiments.
Preparation of P9 bacterial suspension and cultivation of peanut seedlings
LB liquid medium was inoculated with an appropriate amount of P9 strain (1%) and shaken at 150 rpm overnight at 30°C. The next day, the cultures were centrifuged at 5000 × g for 10 min, the supernatant was discarded, and the bacteria were rinsed twice with sterile water. The bacteria were collected and suspended in sterile water to prepare a 108 CFU/mL P9 suspension.
Peanut seeds were sterilized with 20% H2O2 solution for 20 min and rinsed repeatedly with sterile water. After soaking in sterile water for 12 h, the seeds were placed in a Petri dish with a double layer of sterile moist filter paper and germinated in a light incubator (16/8 h photo-period) at 28°C for about 3 days. Germinated peanut seedlings were selected and planted in plastic pots containing 600 g sterile soil for routine management.
Experimental design and treatments
The experiment setup was a pot experiment. Samples were divided into the control group (CK), inoculation group (P9), drought-stress group (DR), and inoculation+drought-stress group (P9+DR), with 24 replicates for each treatment. The inoculation treatment was performed when the first true leaves of peanut seedlings were fully expanded. P9 and P9+DR groups were irrigated with bacterial suspension (20 mL) every 3 days, whereas CK and DR groups were irrigated with the same volume of sterile water. After 30 days of irrigation, DR and P9+DR groups were subjected to drought stress without irrigation, and watering was resumed after 12 days of drought. CK and P9 groups continued to be watered or inoculated every 3 days. From the beginning of the drought treatment until 7 days after re-watering, we picked randomly 4 plants to measure the following indexes every 3 days, including the growth and physiological indexes, antioxidant enzyme activities, and the stomatal characteristics of leaves.
Determination of plant growth indexes, physiological indexes and antioxidant enzyme activities
Peanut seedlings were collected, washed with sterile water, and dried with filter paper. Growth indicators, such as plant height, fresh weight, and root length and weight were measured. Physiological indexes assayed included chlorophyll content, proline content, malondialdehyde (MDA) content, leaf relative water content, soil relative water content, H2O2 content, and ROS levels. The chlorophyll content was determined by the ethanol extraction colorimetric method (Wang et al., 2009). The proline content was determined by the acid hydrate ninhydrin colorimetric method (Wang et al., 2007). The MDA content was determined by the thiobarbituric acid colorimetric method (Song et al., 2011). The relative water content of leaves was determined by the saturated water content method (Cohen et al., 2009), and the relative water content of the soil was determined by the gravimetry method (Dobriyal et al., 2012). ROS analysis was performed by nitro-blue tetrazolium (NBT) staining (Alexander et al., 2020; Gowtham et al., 2020). Antioxidant enzyme activities, including superoxide dismutase (SOD) activity, peroxidase (POD) activity, and catalase (CAT) activity, and the H2O2 content were measured using relevant kits obtained from Comin Biotechnology Co., Ltd. (Suzhou, China). The determination of SOD activity was based on the NBT colorimetric method (García-Triana et al., 2010), and POD activity was measured by the guaiacol colorimetric method (Markkola et al., 2002). CAT activity was determined by the UV absorption method, and the H2O2 content was measured by the titanium sulfate colorimetric method (Sima et al., 2011).
Analysis of stomatal characteristics of peanut leaves
Peanut leaves were fixed and decolorized in anhydrous ethanol; the chlorophyll was then removed and transferred to a mixture of 95% ethanol and NaClO (6%–14%) in a 1:1 (v/v) ratio. After the leaves became transparent, they were transferred to clean water, placed on a glass slide with a few drops of distilled water, and covered with a coverslip (Sultana et al., 2021). They were then observed and photographed using a BX53 microscope (Olympus Corp., Tokyo, Japan). Five random photographs were taken under a 40 objective to measure stomatal density (mean number of stomata/field-of-view area), length (length of guard cells), width (the width of guard cells), and aperture (width of the stomatal aperture) using cellSens Standard software (Olympus Corp.).
Data were analyzed using IBM SPSS Statistics 20.0. Duncan’s method was used for the comparison of mean values. Excel was used for data processing and mapping. The least significant difference (LSD) test was used for multiple comparisons (p < 0.05). Data were represented as means ± standard errors.
Effects of P9 inoculation on the growth of peanut under drought stress and after re-watering
The effects of T. tyrosinosolvens P9 strain inoculation on the growth of peanut seedlings under normal conditions, drought stress, and after re-watering were analyzed (Fig. 1; Table 1). Under normal water conditions, plant height and the fresh weight of inoculated peanuts were significantly higher than the controls (p < 0.05). The root length and weight were also higher than the controls to different extents. Under drought stress, peanut growth was inhibited during extended drought. On day 12 of the drought condition, the plants showed obvious wilting due to severe water shortage; however, the growth indexes of P9+DR plants were significantly better than those of the DR group (p < 0.05). Compared with DR treatment, the heights of P9+DR plants were 23.06%–59.97% greater, fresh weight increased by 26.38%–47.13%, root length improved by 17.39%–39.63%, and root weight increased by 16.67%–61.82%. These observations suggest that P9 inoculation can promote nutritional growth and root development in peanut under drought stress. After re-watering, the growth status of the DR group peanut plants gradually recovered, while the recovery efficiency of P9+DR plants was better, and recovery occurred more quickly. Further, the plant height, fresh weight, and root weight of P9+DR plants were significantly greater than those of DR plants (p < 0.05).
Figure 1: Effects of Tsukamurella tyrosinosolvens P9 inoculation on the growth of peanut under drought stress and after re-watering. (a) Drought stress 0 d; (b) Drought stress 12 d; (c) Re-watering 7 d. From left to right is CK (control peanut), P9 (P9-inoculated peanut), DR (drought-treated peanut), and P9+DR (P9-inoculated peanut under drought treatment), respectively. Each group had four replicates.
Effect of P9 inoculation on physiological indexes of peanut under drought stress and after re-watering
The physiological indexes of the plants were analyzed after different treatments (Figs. 2 and 3). Under normal water conditions, P9 inoculation had no significant effect on the soil relative water and MDA contents of peanut seedlings; but, leaf relative water, chlorophyll, and proline contents were significantly higher than those of CK plants. The H2O2 content was significantly lower than in CK plants (p < 0.05). Under drought stress, while the soil water content decreased sharply, leaf water and chlorophyll contents decreased successively, and the contents of proline, MDA, and H2O2 increased sharply with the extension of drought stress. The changes in the physiological indexes of inoculated plants showed different characteristics: the soil water, leaf water, and chlorophyll contents of the P9+DR group were significantly higher than those of the DR group while the proline, MDA, and H2O2 contents were significantly lower than those of the DR group (p < 0.05) However, the proline content was not significantly different from that of the DR group after 3 days of drought. To elaborate, during 0–12 days of drought, compared with the DR group, the soil water content of the P9+DR group increased from 0.48%–32.77%, the leaf water content increased by 6.56%–42.97%, the chlorophyll content increased by 31.61%–38.78%. However, the MDA content decreased by 26.83%–49.03%, and the H2O2 content decreased by 24.24%–45.41%. It showed that P9 inoculation reduced the loss of water from plants and soil under stress, and alleviated the damage to seedlings induced by drought stress. After 7 days of re-watering, the soil water content returned to normal, leaf water and chlorophyll contents increased while the contents of proline, MDA, and H2O2 decreased significantly. However, the leaf water content, chlorophyll content, and proline content of P9+DR plants were higher, while MDA and H2O2 contents were lower than the DR group. Therefore, inoculation with P9 could reverse the effects of drought stress on peanut more quickly after re-watering.
Figure 2: Effect of Tsukamurella tyrosinosolvens P9 inoculation on the relative water content of peanut leaves and soil under drought stress and after re-watering. For each sample, different lowercase letters after the numbers in the same column represent significant differences between treatments at the same time points (p < 0.05). Values are given as means ± S.E.
Figure 3: Effect of Tsukamurella tyrosinosolvens P9 inoculation on physiological indexes of peanut under drought stress and after re-watering. For each sample, different lowercase letters after the numbers in the same column represent significant differences between treatments at the same time points (p < 0.05). Values are given as means ± S.E.
Effects of P9 inoculation on ROS accumulation in peanut leaves under drought stress and after re-watering
Oxygen free radicals can convert NBT into a blue or brown precipitate; thus, it can be used to visually locate and observe the sites where O2− is produced and accumulated (Alexander et al., 2020). The results of the NBT staining showed that O2− production increased significantly under drought stress, and the brown deposition in leaves became more obvious with the extension of drought which decreased after re-watering (Fig. 4). Compared with drought stress alone, the accumulation of O2− in inoculated plants was significantly lower, also indicating that the P9 inoculation alleviated the oxidative damage caused by drought stress, which was consistent with the results of the H2O2 content determination.
Figure 4: Effects of Tsukamurella tyrosinosolvens P9 inoculation on ROS accumulation in peanut leaves during different drought stress times and after re-watering. In vivo localization of ROS in peanut leaves as revealed by nitro-blue tetrazolium (NBT) staining. From left to right are CK (control peanut), P9 (P9-inoculated peanut), DR (drought-treated peanut), and P9+DR (P9-inoculated peanut under drought treatment), respectively.
Effects of P9 inoculation on antioxidant enzyme activities in peanut under drought stress and after re-watering
As shown in Table 2, under normal water conditions, there was no significant difference in the enzyme activities of SOD, CAT, and POD between inoculated and uninoculated plants. Under drought stress, while antioxidant enzyme activities increased, with the prolongation of drought stress, the changes in the activities of the three enzymes differed. The activities of SOD and POD increased gradually, with a significant change in POD activity, while CAT activity increased first and then decreased. During days 3–12 of drought stress, the CAT activity of the P9+DR group was significantly higher than that of the DR group. The SOD activity was also significantly higher, except after 6 d of drought stress. However, the POD activity changed only slightly and was only significantly higher than the DR group after day 9 of drought treatment. After re-watering, the activities of SOD, POD, and CAT in the P9+DR and DR groups returned to normal levels, and there was no significant difference between the groups.
Effect of P9 inoculation on the stomatal status of peanut under drought stress and after re-watering
The stomatal status of peanut leaves was analyzed under normal conditions, drought stress, and post-drought re-watering (Table 3). Under normal conditions, while stomatal density, length, width, and aperture were constant, these indexes were significantly higher in the P9 group than in the CK group (p < 0.05). Under drought stress, stomatal length, width, and aperture decreased, whereas stomatal density increased, and the changes were more pronounced with longer drought stress. However, the stomatal length, width, and aperture of P9+DR plants were significantly higher than those of the DR group (p < 0.05). Compared with the DR group, stomatal length, width, and aperture were increased by 11.81%–21.90%, 13.60%–28.75%, and 18.36%–39.17%, respectively, in the P9+DR group during drought treatment. The effect of stress on stomatal density was slightly different. In the early stages (0–3 d) of drought stress, the stomatal densities of inoculated plants were significantly higher than those of the DR group, while in the late stages of drought (9–12 d), the stomatal density of the P9+DR group was significantly lower than that of the DR group (p < 0.05). After 7 days of re-watering, the stomatal status gradually returned to its unstressed status, and the stomatal length, width, and aperture of P9+DR plants were significantly higher than those of the uninoculated group (p < 0.05). The stomatal density did not differ significantly between the two treatments.
In this study, under normal water conditions, P9 inoculation in peanut plants improved their growth indexes, the relative water content, chlorophyll content, proline content. While the stomatal indexes were significantly higher than in the CK group; the contents of MDA and H2O2 of the inoculated plants were significantly lower than in the CK group (p < 0.05). The results showed that inoculation with T. tyrosinosolvens P9 significantly improved the physiological indexes of peanut, reduced the level of stress, and promoted seedling growth. Another study showed that inoculation with Bacillus pummilus and B. mycoides could improve photosynthesis, water use efficiency, and the antioxidant enzyme activity of runner bean plants and promote plant growth (Stefan et al., 2013). In another report, inoculation with B. velezensis could improve the stomatal aperture and density of pepper, resulting in a greater CO2 absorption rate and enhanced photosynthesis (Sevirasari et al., 2022). Similar results were obtained for Klebsiella variicola-, Raoultella planticola-, and Pseudomonas fluorescens-inoculated maize; B. subtilis-, P. fluorescens-, and P. putida-inoculated mint; and B. amyloliquefaciens-inoculated wheat (del Rosario Cappellari et al., 2015; Gou et al., 2015; Danish and Zafar-ul-Hye, 2019).
Drought affects plant growth and metabolism. Generally, when the soil moisture is 55%–60%, 45%–50%, and 35%–40%, plants suffer from mild, moderate, and severe water stress, respectively, which can lead to plant growth arrest and even death (Yuan et al., 2016). PGPRs were shown to improve the stress resistance of plants. For example, inoculation with P. putida increased the total root length, stem length, and biomass of sunflower seedlings under water stress (Sandhya et al., 2009). Inoculation with B. licheniformis increased the dry weights of maize roots and stems under stress (Akhtar et al., 2020) and Burkholderia seminalis inoculation increased the biomasses of tomato and pepper under stress (Tallapragada et al., 2016). On the basis of phylogenetic profiling, the dominant phylum of drought-adaptive bacteria has been observed to be Proteobacteria with 31 species, of which 16 species belonged to the genus Pseudomonas followed by Enterobacter, Serratia, Acinetobacter, Burkholderia, etc. The second dominant phylum included Firmicutes, of which most belonged to the genus Bacillus. Another phylum reported included Actinobacteria of which most members belonged to the genus Streptomyces (Kour and Yadav, 2022).
It was reported that Streptomyces sp. inoculation improved the growth and drought resistance of plants such as chickpea and tomato (Sreevidya et al., 2016; Koçak, 2019). Several studies have shown that the ecological distribution of actinomycetes is negatively correlated with soil moisture, and they tend to be dominant in drought environments because they are strongly drought-tolerant (Khamna et al., 2010). In this study, we found that the growth of peanut was inhibited when the period of drought was extended, however, seedlings inoculated with T. tyrosinosolvens P9 fared significantly better than the DR group. The plant height, fresh weight, and root length and weight were all significantly higher in these inoculated plants (p < 0.05). The colonization by PGPRs is a key factor influencing their growth-promoting function in plants (Mohanram and Kumar, 2019). We found that T. tyrosinosolvens P9 (a member of actinobacteria) could stably colonize the root tips of peanut seedlings, accumulate in root hairs, migrate to stem tissues to then exert their growth-promoting effects on peanut (Li et al., 2022b). All these results provided a theoretical basis for the application of this strain to improve the drought resistance of peanut.
Under drought stress, an imbalance between ROS production and clearance occurs in plants, which leads to an excessive accumulation of ROS. The free radical oxygen could convert unsaturated fatty acids into lipid peroxidation products, such as MDA, which further damage the structure and function of the cell membrane. Therefore, MDA is an important indicator of the degree of damage to membrane lipids and stability caused by ROS (Das and Roychoudhury, 2014). H2O2 is a common ROS and its accumulation can lead to cell membrane damage (Ahmed et al., 2009). In this study, the contents of MDA and H2O2 were significantly increased under drought stress. However, P9 inoculation significantly reduced both MDA and H2O2 contents (p < 0.05). NBT staining also clearly indicated more obvious brown depositions and the accumulation of O2− was even greater in the leaves of the DR group. This suggests that the P9 strain can alleviate oxidative damage caused by drought stress. Batool et al. (2020) also found that inoculation with B. subtilis could enhance the ability to scavenge ROS and reduce the accumulation of ROS and MDA that reduced membrane damage, and enhanced drought resistance.
PGPRs can improve the stress resistance of plants, which is closely related to their ability to regulate plant physiological changes. Soil water deficiency is an important factor limiting plant growth. PGPRs can effectively adhere to soil and improve soil water status. Additionally, IAA-producing PGPR strains can stimulate root development, and increase water absorption (Sandhya et al., 2009; Marasco et al., 2013; Luo et al., 2019). Kubi et al. (2021) reported that plant root inoculation with IAA-secreting P. psychrotolerans promoted the growth of Zea mays. As a major plant hormone, IAA was involved in plant cell division and differentiation, germination, and most importantly root growth (Etesami and Beattie, 2017). In this report, the root length and root weight of the P9+DR group were significantly higher than those of the DR group. Hence, the inoculation of the P9 strain obviously improved the root development and water uptake under drought-stress conditions. This improvement may be related to the ability of the microbe to secre IAA (37.26 g/mL) (Zhang et al., 2021). Marulanda et al. (2006) also reported that plants treated with Bacillus thuringiensis had better root biomass, including much more root branch numbers and root surface areas, and could absorb more water from the soil.
Leaf water content is an important indicator of the degree of water deficiency, and higher relative water content indicates that a plant is more adaptable to environmental stress (Moshelion et al., 2015). During drought stress, inoculation with P. putida improved the water status of chickpea leaves and alleviated membrane damage and oxidative stress (Tiwari et al., 2016). In this study, when drought was extended, the water contents of the soil and leaves decreased significantly, but these indexes were significantly higher in the P9 inoculated plants (p < 0.05). Obviously, PGPR improves the drought resistance of plants by delaying plant dehydration, increasing their water content, and maintaining proper cellular water status (Khan et al., 2020). Similar results have also been reported for Bacillus sp., Pseudomonas sp. and Enterobacter sp. (Niu et al., 2018; He et al., 2021). Stomatal regulation is the initial response of plants to drought stress; in particular, plants reduce water loss by regulating stomatal characteristics, including stomatal morphology (stomatal size and density) and stomatal movement (stomatal aperture) (McAdam and Brodribb, 2012; Daszkowska-Golec and Szarejko, 2013). Plants grown under water deficit conditions have a lower stomata conductance to facilitate the conservation of water (Hayat et al., 2010). A decline in leaf water content will lead to a decrease in stomatal aperture and even closure (Casson and Hetherington, 2010). Under drought stress, the stomatal density of peanut leaves significantly increased, while stomatal length, width, and aperture all significantly decreased (p < 0.05). However, the stomatal density of P9-inoculated plants was higher at the beginning of the stress period but lower than the DR group by the end of the stress period (9–12 d). The stomatal length, width, and aperture were significantly higher than the DR group (p < 0.05). This is likely due to the following hypothesis: under drought conditions, the leaf area shrinks due to an obvious decrease in leaf water content. Thus, the stomatal density increases due to an increase in stomata number per unit area.
The leaf size has been negatively correlated with stomatal density (Doheny-Adams et al., 2012; Liu et al., 2012). However, peanut adapts to drought by reducing stomatal length, width, and aperture to maintain lower transpiration rates and reduce water loss. A similar response mechanism is found in vegetable crops, such as eggplant, corn, and wheat (Fu et al., 2013; Zhao et al., 2015; Wang et al., 2016), as well as Leymus chinensis, Arabidopsis thaliana, and cattail (Xu and Zhou, 2008; Doheny-Adams et al., 2012; Cruz et al., 2019). These results all show that more, smaller stomata are a response to water stress. Furthermore, higher ROS levels could lead to increased ABA accumulation, which leads to stomatal closure (Pirasteh-Anosheh et al., 2016). These results are similar to those of the present study, which showed that ROS accumulation was higher in uninoculated plants, and therefore leaf stomatal size and aperture were significantly decreased. In addition, the leaf water contents of inoculated plants were still 73.83%–80.24% after 9–12 d of stress, and peanut plants do not need to reduce transpiration by closing or shrinking the stomata. Hence, inoculation with PGPR could increase the leaf area by increasing soil and leaf water contents during drought stress, which promoted stomatal opening and relieved the inhibition of photosynthesis (Liu et al., 2019a; Bashir and Naz, 2020; Suryanti and Umami, 2020). Accordingly, although the chlorophyll content of peanut significantly decreased under drought stress: with its content in the P9+DR group being significantly higher than those of the DR group (p < 0.05). Stress can lead to the degradation of chlorophyll and a decrease in plant photosynthetic capacity (Kawamitsu et al., 2000). However, inoculation with Acinetobacter sp., Serratia marcescens, and Pseudomonas sp. reduced the damage to photosynthetic organs, increased the chlorophyll content and the photosynthetic rate of plants to compensate for the adverse effect of drought (Abbasi et al., 2013; Liu et al., 2019b; Khan and Singh, 2021). After re-watering, the stomatal status of the plants gradually returned to normal and bacterial inoculation promoted the rapid recovery of the density, size, and aperture of stomata and the photosynthetic function in peanut. Similar results have been reported in elderberry inoculated with Acinetobacter calcoaceticus and sorghum inoculated with Bacillus sp. (Liu et al., 2019b; Santana et al., 2020).
To reduce the toxic effect of drought, plants eliminate the excess accumulated ROS by regulating antioxidant enzymes and non-enzymatic antioxidants. The enzymatic antioxidant defense system mainly includes SOD, POD, and CAT: O2− produced under stress can be converted to H2O2 and O2 by SOD, while CAT and POD convert H2O2 to H2O, thus reducing ROS damage to plant cells (Sharma et al., 2012). In this study, the activities of SOD and POD increased, especially that of POD, under drought stress, while the activity of CAT initially increased and then decreased. Plants often resist oxidative damage by increasing the activity of antioxidant enzymes under stress (Jin et al., 2015). For example, the CAT activity of ramie increased first and then decreased during prolonged stress, while the activities of SOD and POD increased gradually, This indicates that the changes in enzyme activity were closely related to the intensity and duration of stress (Huang et al., 2013). As the large amount of H2O2 produced by drought stress inhibits CAT activity, its activity decreased during the later stage of drought. However, the increase in SOD and POD activities, especially POD, may compensate for such decreased CAT activity to some extent (Pan et al., 2006). However, the antioxidant enzyme activity of inoculated peanut was higher than that of the DR group, which indicated that strain P9 was able to activate the antioxidant enzyme system of peanut. Therefore, inoculation with strain P9 significantly reduced the accumulation of O2− and H2O2 during drought stress by increasing the activities of CAT, POD, and SOD and enhancing the drought resistance of peanut. A study by Gupta et al. (2015) found increased CAT and SOD activities in leaves of Helianthus annuus seedlings inoculated with Azotobacter chroococcum and Bacillus polymyxa under water deficit conditions. Further, similar results also have been reported in other studies (Ghorbanpour et al., 2013; Sarma and Saikia, 2014; Gusain et al., 2015; Zhang et al., 2020).
Osmotic regulation is another important protective mechanism against stress in plants. As a common osmotic regulator, a large amount of proline is essential to reduce oxidative stress and reduce damage to the photosystem and chlorophyll during stress (Hayat et al., 2012; Shan et al., 2015). In this study, under drought stress, the proline content of peanut significantly increased, and the increase in proline content was lower in the late stage of stress in the P9+DR group compared to the DR group. The increment in proline by PGPR under drought has been reported in cucumber, potato plants, and great millet to improve their survival and drought tolerance (Vanderlinde et al., 2010; Wang et al., 2012; Gururani et al., 2013; Kour et al., 2020). However, there are different results in some reports. For example, Naveed et al. (2014) found that the proline content of wheat increased with worsening drought, whereas inoculation with Burkholderia phytofirmans reduced proline accumulation. Proline accumulation also decreased in plants inoculated with Klebsiella sp., Enterobacter ludwigii, and Flavobacterium sp. (Gontia-Mishra et al., 2016). However, other studies showed that proline accumulation is a symptom of stress injury instead of serving as an indicator of stress tolerance. The accumulation level is usually positively correlated with the degree of stress in cells (Monreal et al., 2007). In cultivars with weak drought resistance, proline accumulation is higher under stress (Sundaresan and Sudhakaran, 1995; Silvente et al., 2012). Therefore, in our work, the accumulation of proline was reduced in inoculated peanuts in the late drought period, explaining why strain P9 improved the stress due to water shortage and alleviated photosynthetic damage.
After re-watering, the soil water content returned to the normal level in the DR group, but the leaf water content remained lower, and the contents of MDA and H2O2 were still significantly higher than those of the CK plants. Thus, although the growth conditions of the peanut plants gradually recovered, the plants did not completely recover from the stress and had some degree of oxidative damage. As for the P9+DR group, after re-watering, while the water content, chlorophyll content, and proline content were significantly higher than the DR group, the H2O2 content was significantly lower than the latter (p < 0.05), and the growth of peanut plants improved. Bresson et al. (2014) also found that inoculation with Phyllobacterium brassicacearum not only maintained the water content of A. thaliana leaves under stress but also aided plant recovery after re-watering. Similar studies have been reported in elderbush inoculated with A. calcoaceticus and wheat inoculated with Alternaria alternata (Liu et al., 2019b; Qiang et al., 2019). Therefore, strain P9 conferred drought tolerance to the re-watered plants, which was beneficial to photosynthesis and biomass accumulation under stress and after re-watering.
In this paper, the effects of the PGPR strain T.tyrosinosolvens P9 on peanut growth and drought resistance were investigated. During drought stress, the water content of the soil and leaves and the chlorophyll content decreased with the length of the drought period. Although the effects of drought were alleviated in the plants by increasing their proline content and antioxidant enzyme activities, the MDA and H2O2 contents increased sharply with the extension of the drought, and the growth of peanut plants was inhibited. Compared with the DR group, inoculation with the P9 strain significantly increased the water contents of the soil and leaves; chlorophyll content, and the length, width, and aperture of the stomata (p < 0.05). The stomatal density decreased at the later stage of drought stress; the activities of CAT and SOD (except for 6 d of stress) were significantly increased, and the contents of MDA and H2O2 in inoculated plants were significantly lower than those in the DR group (p < 0.05). Accordingly, during drought, the growth indexes of inoculated seedlings were significantly better than those of uninoculated plants (p < 0.05). In addition, inoculated peanut plants resumed growing faster after the drought stress. Therefore, Tsukamurella tyrosinosolvens P9 inoculation could reduce the effects of stress, improve peanut drought resistance, promote seedling growth by improving plant water status, photosynthetic performance, and stomatal status, while activating the antioxidant enzyme system, reducing membrane lipid peroxidation and cell damage caused by stress. Our results provide a theoretical foundation for the further application of various PGPR strains and a new approach to improving drought resistance in agricultural plants.
Acknowledgement: We thank Candace Webb, Ph.D., from Liwen Bianji (Edanz) (www.liwenbianji.cn) for editing the English text of the draft of this manuscript.
Funding Statement: This work was supported by the National Natural Science Foundation of China under Grant 31760030 and 32060028, and the Science and Technology Planning Project of Guizhou Province under Grant [2022]009.
Author Contributions: Lizhen Han is the corresponding author while Changmei Long, Tingting Yang, and Yujie Han are Lizhen Han’s graduate students. Changmei Long designed the experiment and wrote the manuscript. The three students performed all experiments. All authors read and approved the final manuscript.
Availability of Data and Materials: All data generated or analyzed during this study are included in this published article.
Ethics Approval: Not applicable.
Conflicts of Interest: The authors declare that they have no conflicts of interest to report regarding the present study.
References
Abbasi S, Zahedi H, Sadeghipour O, Akbari R (2013). Effect of plant growth promoting rhizobacteria (PGPR) on physiological parameters and nitrogen content of soybean grown under different irrigation regimes. Research Crops 14: 798–803. https://www.researchgate.net/publication/286171090 [Google Scholar]
Abdelaal K, AlKahtani M, Attia K, Hafez Y, Király L, Künstler A (2021). The role of plant growth-promoting bacteria in alleviating the adverse effects of drought on plants. Biology 10: 520. https://doi.org/10.3390/biology10060520 [Google Scholar] [PubMed] [CrossRef]
Ahmed CB, Rouina BB, Sensoy S, Boukhris M, Abdallah FB (2009). Changes in gas exchange, proline accumulation and antioxidative enzyme activities in three olive cultivars under contrasting water availability regimes. Environmental and Experimental Botany 67: 345–352. https://doi.org/10.1016/j.envexpbot.2009.07.006 [Google Scholar] [CrossRef]
Akhtar SS, Amby DB, Hegelund JN, Fimognari L, Grobkinsky DK, Westergaard JC (2020). Bacillus licheniformis FMCH001 increases water use efficiency via growth stimulation in both normal and drought conditions. Frontiers in Plant Science 11: 297. https://doi.org/10.3389/fpls.2020.00297 [Google Scholar] [PubMed] [CrossRef]
Alexander A, Singh VK, Mishra A (2020). Halotolerant PGPR Stenotrophomonas maltophilia BJ01 induces salt tolerance by modulating physiology and biochemical activities of Arachis hypogaea. Frontiers in Microbiology 11: 568289. https://doi.org/10.3389/fmicb.2020.568289 [Google Scholar] [PubMed] [CrossRef]
Bashir T, Naz S (2020). Plant growth promoting rhizobacteria in combination with plant growth regulators attenuate the effect of drought stress. Pakistan Journal of Botany 52: 783–793. https://doi.org/10.3389/fpls.2022.875774 [Google Scholar] [PubMed] [CrossRef]
Batool T, Ali S, Seleiman MF, Naveed NH, Ali A et al. (2020). Plant growth promoting rhizobacteria alleviates drought stress in potato in response to suppressive oxidative stress and antioxidant enzymes activities. Scientific Reports 10: 1–19. https://doi.org/10.1038/s41598-020-73489-z [Google Scholar] [PubMed] [CrossRef]
Bouskill NJ, Lim HC, Borglin S, Salve R, Wood TE, Silver WL, Brodie EL (2013). Pre-exposure to drought increases the resistance of tropical forest soil bacterial communities to extended drought. The ISME Journal 7: 384–394. https://doi.org/10.1038/ismej.2012.113 [Google Scholar] [PubMed] [CrossRef]
Bresson J, Vasseur F, Dauzat M, Labadie M, Varoquaux F, Touraine B, Vile D (2014). Interact to survive: Phyllobacterium brassicacearum improves Arabidopsis tolerance to severe water deficit and growth recovery. PLoS One 9: e107607. https://doi.org/10.1371/journal.pone.0107607 [Google Scholar] [PubMed] [CrossRef]
Casson SA, Hetherington AM (2010). Environmental regulation of stomatal development. Current Opinion in Plant Biology 13: 90–95. https://doi.org/10.1016/j.pbi.2009.08.005 [Google Scholar] [PubMed] [CrossRef]
Cohen AC, Travaglia CN, Bottini R, Piccoli PN (2009). Participation of abscisic acid and gibberellins produced by endophytic Azospirillum in the alleviation of drought effects in maize. Botany 87: 455–462. https://doi.org/10.1139/B09-023 [Google Scholar] [CrossRef]
Collin F (2019). Chemical basis of reactive oxygen species reactivity and involvement in neurodegenerative diseases. International Journal of Molecular Sciences 20: 2407. https://doi.org/10.3390/ijms20102407 [Google Scholar] [PubMed] [CrossRef]
Cruz YDC, Scarpa ALM, Pereira MP, de Castro EM, Pereira FJ (2019). Growth of Typha domingensis as related to leaf physiological and anatomical modifications under drought conditions. Acta Physiologiae Plantarum 41: 1–9. https://doi.org/10.1007/s11738-019-2858-1 [Google Scholar] [CrossRef]
Danish S, Zafar-ul-Hye M (2019). Co-application of ACC-deaminase producing PGPR and timber-waste biochar improves pigments formation, growth and yield of wheat under drought stress. Scientific Reports 9: 1–13. https://doi.org/10.1038/s41598-019-42374-9 [Google Scholar] [PubMed] [CrossRef]
Das K, Roychoudhury A (2014). Reactive oxygen species (ROS) and response of antioxidants as ROS-scavengers during environmental stress in plants. Frontiers in Environmental Science 2: 53. https://doi.org/10.3389/fenvs.2014.00053 [Google Scholar] [CrossRef]
Daszkowska-Golec A, Szarejko I (2013). Open or close the gate-stomata action under the control of phytohormones in drought stress conditions. Frontiers in Plant Science 4: 138. https://doi.org/10.3389/fpls.2013.00138 [Google Scholar] [PubMed] [CrossRef]
del Rosario Cappellari L, Santoro MV, Reinoso H, Travaglia C, Giordano W, Banchio E (2015). Anatomical, morphological, and phytochemical effects of inoculation with plant growth-promoting rhizobacteria on peppermint (Mentha piperita). Journal of Chemical Ecology 41: 149–158. https://doi.org/10.1007/s10886-015-0549-y [Google Scholar] [PubMed] [CrossRef]
Ding H, Zhang ZM, Zhang GC, Xu Y, Guo Q, Qin FF, Dai LX (2022). Nitrogen application improved peanut yield and nitrogen use efficiency by optimizing root morphology and distribution under drought stress. Chilean Journal of Agricultural Research 82: 256–265. https://doi.org/10.4067/S0718-58392022000200256 [Google Scholar] [CrossRef]
Dobriyal P, Qureshi A, Badola R, Hussain SA (2012). A review of the methods available for estimating soil moisture and its implications for water resource management. Journal of Hydrology 458: 110–117. https://doi.org/10.1016/j.jhydrol.2012.06.021 [Google Scholar] [CrossRef]
Doheny-Adams T, Hunt L, Franks PJ, Beerling DJ, Gray JE (2012). Genetic manipulation of stomatal density influences stomatal size, plant growth and tolerance to restricted water supply across a growth carbon dioxide gradient. Philosophical Transactions of the Royal Society B: Biological Sciences 367: 547–555. https://doi.org/10.1098/rstb.2011.0272 [Google Scholar] [PubMed] [CrossRef]
Etesami H, Beattie GA (2017). Plant-microbe interactions in adaptation of agricultural crops to abiotic stress conditions. In: Kumar V, Kumar M, Sharma S, Prasad R, eds. Porbiotics and Plant Health, pp. 163–200, Singapore: Springer. [Google Scholar]
Fu QS, Yang RC, Wang HS, Zhao B, Zhou CL, Ren SX, Guo YD (2013). Leaf morphological and ultrastructural performance of eggplant (Solanum melongena L.) in response to water stress. Photosynthetica 51: 109–114. https://doi.org/10.1007/s11099-013-0005-6 [Google Scholar] [CrossRef]
García-Triana A, Zenteno-Savín T, Peregrino-Uriarte AB, Yepiz-Plascencia G (2010). Hypoxia, reoxygenation and cytosolic manganese superoxide dismutase (cMnSOD) silencing in Litopenaeus vannamei: Effects on cMnSOD transcripts, superoxide dismutase activity and superoxide anion production capacity. Developmental & Comparative Immunology 34: 1230–1235. https://doi.org/10.1016/j.dci.2010.06.018 [Google Scholar] [PubMed] [CrossRef]
Ghorbanpour M, Hatami M, Khavazi K (2013). Role of plant growth promoting rhizobacteria on antioxidant enzyme activities and tropane alkaloid production of Hyoscyamus niger under water deficit stress. Turkish Journal of Biology 37: 350–360. https://doi.org/10.3906/biy-1209-12 [Google Scholar] [CrossRef]
Gontia-Mishra I, Sapre S, Sharma A, Tiwari S (2016). Amelioration of drought tolerance in wheat by the interaction of plant growth-promoting rhizobacteria. Plant Biology 18: 992–1000. https://doi.org/10.1111/plb.12505 [Google Scholar] [PubMed] [CrossRef]
Gou W, Tian L, Ruan Z, Zheng P, Chen FC et al. (2015). Accumulation of choline and glycinebetaine and drought stress tolerance induced in maize (Zea mays) by three plant growth promoting rhizobacteria (PGPR) strains. Pakistan Journal of Botany 47: 581–586. http://ir.iswc.ac.cn/handle/361005/7633 [Google Scholar]
Gowtham HG, Singh B, Murali M, Shilpa N, Prasad M, Aiyaz M, Amruthesh KN, Niranjana SR (2020). Induction of drought tolerance in tomato upon the application of ACC deaminase producing plant growth promoting rhizobacterium Bacillus subtilis Rhizo SF 48. Microbiological Research 234: 126422. https://doi.org/10.1016/j.micres.2020.126422 [Google Scholar] [PubMed] [CrossRef]
Gupta G, Parihar SS, Ahirwar NK, Snehi SK, Singh V (2015). Plant growth promoting rhizobacteria (PGPRCurrent and future prospects for development of sustainable agriculture. Journal of Microbial and Biochemical Technology 7: 96–102. https://doi.org/10.1007/978-981-15-6949-4_9 [Google Scholar] [CrossRef]
Gururani MA, Upadhyaya CP, Baskar V, Venkatesh J, Nookaraju A, Park SW (2013). Plant growth-promoting rhizobacteria enhance abiotic stress tolerance in Solanum tuberosum through inducing changes in the expression of ROS-scavenging enzymes and improved photosynthetic performance. Journal of Plant Growth Regulation 32: 245–258. https://doi.org/10.1007/s00344-012-9292-6 [Google Scholar] [CrossRef]
Gusain YS, Singh US, Sharma AK (2015). Bacterial mediated amelioration of drought stress in drought tolerant and susceptible cultivars of rice (Oryza sativa L.). African Journal of Biotechnology 14: 764–773. https://doi.org/10.5897/AJB2015.14405 [Google Scholar] [CrossRef]
Hayat R, Ali S, Amara U, Khalid R, Ahmed I (2010). Soil beneficial bacteria and their role in plant growth promotion: A review. Annals of Microbiology 60: 579–598. https://doi.org/10.1007/s13213-010-0117-1 [Google Scholar] [CrossRef]
Hayat S, Hayat Q, Alyemeni MN, Wani AS, Pichtel J, Ahmad A (2012). Role of proline under changing environments: A review. Plant Signaling & Behavior 7: 1456–1466. https://doi.org/10.4161/psb.21949 [Google Scholar] [PubMed] [CrossRef]
He A, Niu SQ, Yang D, Ren W, Zhao LY, Sun YY, Meng LS, Zhao Q, Paréa PW, Zhang JL (2021). Two PGPR strains from the rhizosphere of Haloxylon ammodendron promoted growth and enhanced drought tolerance of ryegrass. Plant Physiology and Biochemistry 161: 74–85. https://doi.org/10.1016/j.plaphy.2021.02.003 [Google Scholar] [PubMed] [CrossRef]
Huang CJ, Zhao SV, Wang LC, Anjum SA, Chen M, Zhou HF, Zou CM (2013). Alteration in chlorophyll fluorescence, lipid peroxidation and antioxidant enzymes activities in hybrid ramie (‘boehmeria nivea L.) under drought stress. Australian Journal of Crop Science 7: 594–599. http://www.cropj.com/huang3003_7_5_2013_594_599.pdf [Google Scholar]
Jin R, Shi HT, Han CY, Zhong B, Wang Q, Chan ZL (2015). Physiological changes of purslane (Portulaca oleracea L.) after progressive drought stress and rehydration. Scientia Horticulturae 194: 215–221. https://doi.org/10.1016/j.scienta.2015.08.023 [Google Scholar] [CrossRef]
Kaushal M, Wani SP (2016). Plant-growth-promoting rhizobacteria: Drought stress alleviators to ameliorate crop production in drylands. Annals of Microbiology 66: 35–42. https://doi.org/10.1007/s13213-015-1112-3 [Google Scholar] [CrossRef]
Kawamitsu Y, Driscoll T, Boyer JS (2000). Photosynthesis during desiccation in an intertidal alga and a land plant. Plant Cell Physiology 41: 344–353. https://doi.org/10.1093/pcp/41.3.344 [Google Scholar] [PubMed] [CrossRef]
Khamna S, Yokota A, Peberdy JF, Lumyong S (2010). Indole-3-acetic acid production by Streptomyces sp. isolated from some Thai medicinal plant rhizosphere soils. Eurasian Journal of BioSciences 4: 23–32. https://doi.org/10.5053/ejobios.2010.4.0.4 [Google Scholar] [CrossRef]
Khan N, Ali S, Tariq H, Latif S, Yasmin H, Mehmood A, Shahid MA (2020). Water conservation and plant survival strategies of rhizobacteria under drought stress. Agronomy 10: 1683. https://doi.org/10.3390/agronomy10111683 [Google Scholar] [CrossRef]
Khan A, Singh AV (2021). Multifarious effect of ACC deaminase and EPS producing Pseudomonas sp. and Serratia marcescens to augment drought stress tolerance and nutrient status of wheat. World Journal of Microbiology and Biotechnology 37: 1–17. https://doi.org/10.1007/s11274-021-03166-4 [Google Scholar] [PubMed] [CrossRef]
Kour D, Rana KL, Yadav AN, Yadav N, Kumar V, Kumar A, Sayyed RZ, Hesham AE, Dhaliwal HS, Saxena AK (2019). Drought-Tolerant Phosphorus-Solubilizing Microbes: Biodiversity and Biotechnological Applications for Alleviation of Drought Stress in Plants, pp. 255–308, Singapore: Springer. [Google Scholar]
Kour D, Rana KL, Yadav AN, Yadav N, Kumar M, Kumar V, Vyas P, Dhaliwal HS, Saxena AK (2020). Microbial biofertilizers: Bioresources and eco-friendly technologies for agriucultural and environmental sustainability. Biocatalalysis and Agricultural Biotechnology 23: 101487. https://doi.org/10.1016/j.bcab.2019.101487 [Google Scholar] [CrossRef]
Kour D, Yadav AN (2022). Bacterial mitigation of drought stress in plants: Current perspectives and future challenges. Current Microbiology 79: 248. https://doi.org/10.1007/s00284-022-02939-w [Google Scholar] [PubMed] [CrossRef]
Koçak FÖ (2019). Identification of Streptomyces strains isolated from Humulus lupulus rhizosphere and determination of plant growth promotion potential of selected strains. Turkish Journal of Biology 43: 391. https://doi.org/10.3906/biy-1906-37 [Google Scholar] [PubMed] [CrossRef]
Kubi HAA, Khan MA, Adhikari A, Imran M, Kang SM, Hamayun M, Lee IJ (2021). Silicon and plant growth-promoting rhizobacteria Pseudomonas psychrotolerans CS51 mitigates salt stress in Zea mays L. Agriculture 11: 272. https://doi.org/10.3390/agriculture11030272 [Google Scholar] [CrossRef]
Li C, Yan C, Sun Q, Wang J, Yuan C, Mou Y, Shan S, Zhao X (2022a). Proteomic profiling of Arachis hypogaea in response to drought stress and overexpression of AhLEA2 improves drought tolerance. Plant Biology 24: 75–84. https://doi.org/10.1111/plb.13351 [Google Scholar] [PubMed] [CrossRef]
Li Y, Long CM, Jiang B, Han LZ (2022b). Colonization on the peanuts of two plant-growth promoting rhizobacteria strains and effects on the bacterial community structure of rhizosphere. Biotechnology Bulletin 38: 237. https://doi.org/10.13560/j.cnki.biotech.bull.1985.2021-1577 [Google Scholar] [CrossRef]
Liu FC, Ma HL, Du ZY, Ma BY, Liu XH, Peng L, Zhang WX (2019a). Physiological response of North China red elder container seedlings to inoculation with plant growth-promoting rhizobacteria under drought stress. PLoS One 14: e0226624. https://doi.org/10.1371/journal.pone.0226624 [Google Scholar] [PubMed] [CrossRef]
Liu FC, Ma HL, Peng L, Du ZY, Ma BY, Liu XH (2019b). Effect of the inoculation of plant growth-promoting rhizobacteria on the photosynthetic characteristics of Sambucus williamsii hance container seedlings under drought stress. AMB Express 9: 1–9. https://doi.org/10.1186/s13568-019-0899-x [Google Scholar] [PubMed] [CrossRef]
Liu J, Zhang F, Zhou JJ, Chen F, Wang BS, Xie XZ (2012). Phytochrome B control of total leaf area and stomatal density affects drought tolerance in rice. Plant Molecular Biology 78: 289–300. https://doi.org/10.1007/s11103-011-9860-3 [Google Scholar] [PubMed] [CrossRef]
Luo Y, Wang F, Huang YL, Zhou M, Gao JL, Yan TZ, Sheng HM, An LZ (2019). Sphingomonas sp. Cra20 increases plant growth rate and alters rhizosphere microbial community structure of Arabidopsis thaliana under drought stress. Frontiers in Microbiology 10: 1221. https://doi.org/10.3389/fmicb.2019.01221 [Google Scholar] [PubMed] [CrossRef]
Marasco R, Rolli E, Vigani G, Borin S, Sorlini C, Ouzari H, Zocchi G, Daffonchio D (2013). Are drought-resistance promoting bacteria cross-compatible with different plant models? Plant Signaling & Behavior 8: e26741. https://doi.org/10.4161/psb.26741 [Google Scholar] [PubMed] [CrossRef]
Markkola AM, Tarvainen O, Ahonen-Jonnarth U, Strömmer R (2002). Urban polluted forest soils induce elevated root peroxidase activity in Scots pine (Pinus sylvestris L.) seedlings. Environmental Pollution 116: 273–278. https://doi.org/10.1016/S0269-7491(01)00126-9 [Google Scholar] [PubMed] [CrossRef]
Marulanda A, Barea JM, Azcón R (2006). An indigenous drought-tolerant strain of Glomus intraradices associated with a native bacterium improves water transport and root development in Retama sphaerocarpa. Microbial Ecology 52: 670–678. https://doi.org/10.1007/s00248-006-9078-0 [Google Scholar] [PubMed] [CrossRef]
McAdam SAM, Brodribb TJ (2012). Stomatal innovation and the rise of seed plants. Ecology Letters 15: 1–8. https://doi.org/10.1111/j.1461-0248.2011.01700.x [Google Scholar] [PubMed] [CrossRef]
Mingrou L, Guo S, Ho CT, Bai N (2022). Review on chemical compositions and biological activities of peanut (Arachis hypogeae L.). Journal of Food Biochemistry 46: e14119. https://doi.org/10.1111/jfbc.14119 [Google Scholar] [PubMed] [CrossRef]
Mohanram S, Kumar P (2019). Rhizosphere microbiome: Revisiting the synergy of plant-microbe interactions. Annals of Microbiology 69: 307–320. https://doi.org/10.1007/s13213-019-01448-9 [Google Scholar] [CrossRef]
Monreal JA, Jimenez ET, Remesal E, Morillo-Velarde R, Garcta-Mauriño S, Echevarría C (2007). Proline content of sugar beet storage roots: Response to water deficit and nitrogen fertilization at field conditions. Environmental and Experimental Botany 60: 257–267. https://doi.org/10.1016/j.envexpbot.2006.11.002 [Google Scholar] [CrossRef]
Moshelion M, Halperin O, Wallach R, Oren RAM, Way DA (2015). Role of aquaporins in determining transpiration and photosynthesis in water-stressed plants: Crop water-use efficiency, growth and yield. Plant, Cell & Environment 38: 1785–1793. https://doi.org/10.1111/pce.12410 [Google Scholar] [PubMed] [CrossRef]
Naveed M, Hussain MB, Zahir ZA, Mitter B, Sessitsch A (2014). Drought stress amelioration in wheat through inoculation with Burkholderia phytofirmans strain PsJN. Plant Growth Regulation 73: 121–131. https://doi.org/10.1007/s10725-013-9874-8 [Google Scholar] [CrossRef]
Niu XG, Song LC, Xiao YN, Ge WD (2018). Drought-tolerant plant growth-promoting rhizobacteria associated with foxtail millet in a semi-arid agroecosystem and their potential in alleviating drought stress. Frontiers in Microbiology 8: 2580. https://doi.org/10.3389/fmicb.2017.02580 [Google Scholar] [PubMed] [CrossRef]
Pan Y, Wu LJ, Yu ZL (2006). Effect of salt and drought stress on antioxidant enzymes activities and SOD isoenzymes of liquorice (Glycyrrhiza uralensis Fisch). Plant Growth Regulation 49: 157–165. https://doi.org/10.1007/s10725-006-9101-y [Google Scholar] [CrossRef]
Pirasteh-Anosheh H, Saed-Moucheshi A, Pakniyat H, Pessarakli M (2016). Stomatal responses to drought stress. In: Water Stress and Crop Plants: A Sustainable Approach, vol. 1, pp. 24–40. https://doi.org/10.1002/9781119054450.ch3 [Google Scholar] [CrossRef]
Qiang XJ, Ding JJ, Lin W, Li QZ, Xu CY, Zheng Q, Li YZ (2019). Alleviation of the detrimental effect of water deficit on wheat (Triticum aestivum L.) growth by an indole acetic acid-producing endophytic fungus. Plant and Soil 439: 373–391. https://doi.org/10.1007/s11104-019-04028-7 [Google Scholar] [CrossRef]
Raheem A, Shaposhnikov A, Belimov AA, Dodd IC, Ali B (2018). Auxin production by rhizobacteria was associated with improved yield of wheat (Triticum aestivum L.) under drought stress. Archives of Agronomy and Soil Science 64: 574–587. https://doi.org/10.1080/03650340.2017.1362105 [Google Scholar] [CrossRef]
Sandhya VZAS, Z.A. SK, Grover M, Reddy G, Venkateswarlu BSSS (2009). Alleviation of drought stress effects in sunflower seedlings by the exopolysaccharides producing Pseudomonas putida strain GAP-P45. Biology and Fertility of Soils 46: 17–26. https://doi.org/10.1007/s00374-009-0401-z [Google Scholar] [CrossRef]
Santana SRA, Voltolini TV, Antunes GDR, da Silva VM, Simões WL, Morgante CV, de Freitas ADS, Chaves ARDM, Aidar SDT, Fernandes-Júnior PI (2020). Inoculation of plant growth-promoting bacteria attenuates the negative effects of drought on sorghum. Archives of Microbiology 202: 1015–1024. https://doi.org/10.1007/s00203-020-01810-5 [Google Scholar] [PubMed] [CrossRef]
Sarma RK, Saikia R (2014). Alleviation of drought stress in mung bean by strain Pseudomonas aeruginosa GGRJ21. Plant and Soil 377: 111–126. https://doi.org/10.1007/s11104-013-1981-9 [Google Scholar] [CrossRef]
Sevirasari N, Sulistyaningsih E, Kurniasih B, Suryanti S, Wibowo A, Joko T (2022). Effects of relay intercropping model and application of biological agents on the growth and yield of hot pepper. Ilmu Pertanian (Agricultural Science) 7: 35–46. https://doi.org/10.22146/ipas.69078 [Google Scholar] [CrossRef]
Shan LS, Yang CH, Li Y, Duan YN, Geng DM, Li ZY, Zhang R, Duan GF (2015). Effects of drought stress on root physiological traits and root biomass allocation of Reaumuria soongorica. Acta Ecologica Sinica 35: 155–159. https://doi.org/10.1016/j.chnaes.2015.06.010 [Google Scholar] [CrossRef]
Sharma P, Jha AB, Dubey RS, Pessarakli M (2012). Reactive oxygen species, oxidative damage, and antioxidative defense mechanism in plants under stressful conditions. Journal of Botany 2012: 217037. https://doi.org/10.1155/2012/217037 [Google Scholar] [CrossRef]
Silvente S, Sobolev AP, Lara M (2012). Metabolite adjustments in drought tolerant and sensitive soybean genotypes in response to water stress. PLoS One 7: e38554. https://doi.org/10.1371/journal.pone.0038554 [Google Scholar] [PubMed] [CrossRef]
Sima YH, Yao JM, Hou YS, Wang L, Zhao LC (2011). Variations of hydrogen peroxide and catalase expression in bombyx eggs during diapause initiation and termination. Archives of Insect Biochemistry and Physiology 77: 72–80. https://doi.org/10.1002/arch.20422 [Google Scholar] [PubMed] [CrossRef]
Song H, Wang YS, Sun CC, Wu ML, Peng YL, Deng C, Li QP (2011). Effects of polycyclic aromatic hydrocarbons exposure on antioxidant system activities and proline content in Kandelia candel. Oceanological and Hydrobiological Studies 40: 9–18. https://doi.org/10.2478/s13545-011-0024-5 [Google Scholar] [CrossRef]
Sreevidya M, Gopalakrishnan S, Kudapa H, Varshney RK (2016). Exploring plant growth-promotion actinomycetes from vermicompost and rhizosphere soil for yield enhancement in chickpea. Brazilian Journal of Microbiology 47: 85–95. https://doi.org/10.1016/j.bjm.2015.11.030 [Google Scholar] [PubMed] [CrossRef]
Stefan M, Munteanu N, Stoleru V, Mihasan M (2013). Effects of inoculation with plant growth promoting rhizobacteria on photosynthesis, antioxidant status and yield of runner bean. Romanian Biotechnological Letters 18: 8132–8143. https://www.researchgate.net/profile/Vasile-Stoleru/publication/267865974 [Google Scholar]
Sultana SN, Park H, Choi SH, Jo H, Song JT, Lee JD, Kang YJ (2021). Optimizing the experimental method for stomata-profiling automation of soybean leaves based on deep learning. Plants 10: 2714. https://doi.org/10.3390/plants10122714 [Google Scholar] [PubMed] [CrossRef]
Sundaresan S, Sudhakaran PR (1995). Water stress-induced alterations in the proline metabolism of drought-susceptible and-tolerant cassava (Manihot esculenta) cultivars. Physiologia Plantarum 94: 635–642. https://doi.org/10.1111/j.1399-3054.1995.tb00978.x [Google Scholar] [CrossRef]
Suryanti S, Umami A (2020). Stomata dan trikoma kultivar kedelai anjasmoro selama pemupukan nanosilika dan plant growth promoting rhizobacteria. Vegetalika 9: 343–349. https://doi.org/10.22146/veg.48795 [Google Scholar] [CrossRef]
Tallapragada P, Dikshit R, Seshagiri S (2016). Influence of Rhizophagus spp. and Burkholderia seminalis on the growth of tomato (Lycopersicon esculatum) and bell pepper (Capsicum annuum) under drought stress. Communications in Soil Science and Plant Analysis 47: 1975–1984. https://doi.org/10.1080/00103624.2016.1216561 [Google Scholar] [CrossRef]
Tittabutr P, Piromyou P, Longtonglang A, Noisa-Ngiam R, Boonkerd N, Teaumroong N (2013). Alleviation of the effect of environmental stresses using co-inoculation of mungbean by Bradyrhizobium and rhizobacteria containing stress-induced ACC deaminase enzyme. Soil Science and Plant Nutrition 59: 559–571. https://doi.org/10.1080/00380768.2013.804391 [Google Scholar] [CrossRef]
Tiwari S, Lata C, Chauhan PS, Nautiyal CS (2016). Pseudomonas putida attunes morphophysiological, biochemical and molecular responses in Cicer arietinum L. during drought stress and recovery. Plant Physiology and Biochemistry 99: 108–117. https://doi.org/10.1016/j.plaphy.2015.11.001 [Google Scholar] [PubMed] [CrossRef]
Vanderlinde EM, Harrison JJ, Muszyński A, Carlson RW, Turner RJ, Yost CK (2010). Identification of a novel ABC transporter required for desiccation tolerance, and biofilm formation in Rhizobium leguminosarum bv viciae 3841. FEMS Microbiology Ecology 71: 327–340. https://doi.org/10.1111/j.1574-6941.2009.00824.x [Google Scholar] [PubMed] [CrossRef]
Vurukonda SSKP, Vardharajula S, Shrivastava M, SkZ A (2016). Enhancement of drought stress tolerance in crops by plant growth promoting rhizobacteria. Microbiological Research 184: 13–24. https://doi.org/10.1016/j.micres.2015.12.003 [Google Scholar] [PubMed] [CrossRef]
Wang SY, Hu ZC, Chen YQ, Wu HC, Wang YW, Wu F, Gu FW (2022a). Integration of agricultural machinery and agronomy for mechanised peanut production using the vine for animal feed. Biosystems Engineering 219: 135–152. https://doi.org/10.1016/j.biosystemseng.2022.04.011 [Google Scholar] [CrossRef]
Wang SG, Jia SS, Sun DZ, Hua FAN, Chang XP, Jing RL (2016). Mapping QTLs for stomatal density and size under drought stress in wheat (Triticum aestivum L.). Journal of Integrative Agriculture 15: 1955–1967. https://doi.org/10.1016/S2095-3119(15)61264-3 [Google Scholar] [CrossRef]
Wang Y, Lyu J, Chen D (2022b). Performance assessment of peanut production in China. Acta Agriculturae Scandinavica, Section B-Soil & Plant Science 72: 176–188. https://doi.org/10.1080/09064710.2021.2000632 [Google Scholar] [CrossRef]
Wang M, Wang G, Ji J, Wang JH (2009). The effect of pds gene silencing on chloroplast pigment composition, thylakoid membrane structure and photosynthesis efficiency in tobacco plants. Plant Science 177: 222–226. https://doi.org/10.1016/j.plantsci.2009.04.006 [Google Scholar] [CrossRef]
Wang CJ, Yang W, Wang C, Gu C, Niu DD, Liu HX, Wang YP, Guo JH (2012). Induction of drought tolerance in cucumber plants by a consortium of three plant growth-promoting rhizobacterium strains. PLoS One 7: e52565. https://doi.org/10.1371/journal.pone.0052565 [Google Scholar] [PubMed] [CrossRef]
Wang ZQ, Yuan YZ, Ou JQ, Lin QH, Zhang CF (2007). Glutamine synthetase and glutamate dehydrogenase contribute differentially to proline accumulation in leaves of wheat (Triticum aestivum) seedlings exposed to different salinity. Journal of Plant Physiology 164: 695–701. https://doi.org/10.1016/j.jplph.2006.05.001 [Google Scholar] [PubMed] [CrossRef]
Xu Y, Li Y, Long CM, Han LZ (2022). Alleviation of salt stress and promotion of growth in peanut by Tsukamurella tyrosinosolvens and Burkholderia pyrrocinia. Biologia 77: 2423–2433. https://doi.org/10.1007/s11756-022-01073-z [Google Scholar] [CrossRef]
Xu ZZ, Zhou GS (2008). Responses of leaf stomatal density to water status and its relationship with photosynthesis in a grass. Journal of Experimental Botany 59: 3317–3325. https://doi.org/10.1093/jxb/ern185 [Google Scholar] [PubMed] [CrossRef]
Yuan XK, Yang ZQ, Li YX, Liu Q, Han W (2016). Effects of different levels of water stress on leaf photosynthetic characteristics and antioxidant enzyme activities of greenhouse tomato. Photosynthetica 54: 28–39. https://doi.org/10.1007/s11099-015-0122-5 [Google Scholar] [CrossRef]
Zhang H, Han LZ, Jiang B, Long CM (2021). Identification of a phosphorus-solubilizing Tsukamurella tyrosinosolvens strain and its effect on the bacterial diversity of the rhizosphere soil of peanuts growth-promoting. World Journal of Microbiology and Biotechnology 37: 1–14. https://doi.org/10.1007/s11274-021-03078-3 [Google Scholar] [PubMed] [CrossRef]
Zhang M, Yang L, Hao RQ, Bai XX, Wang Y, Yu X (2020). Drought-tolerant plant growth-promoting rhizobacteria isolated from jujube (Ziziphus jujuba) and their potential to enhance drought tolerance. Plant and Soil 452: 423–440. https://doi.org/10.1007/s11104-020-04582-5 [Google Scholar] [CrossRef]
Zhao WS, Sun YL, Kjelgren R, Liu XP (2015). Response of stomatal density and bound gas exchange in leaves of maize to soil water deficit. Acta Physiologiae Plantarum 37: 1–9. https://doi.org/10.1007/s11738-014-1704-8 [Google Scholar] [CrossRef]
Cite This Article
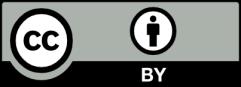
This work is licensed under a Creative Commons Attribution 4.0 International License , which permits unrestricted use, distribution, and reproduction in any medium, provided the original work is properly cited.