Open Access
ARTICLE
Calcitriol induces post-thawed bovine sperm capacitation
Departamento de Biología Molecular, Instituto de Biotecnología Ambiental y Salud (INBIAS), Río Cuarto, 5800, Argentina
* Corresponding Author: NANCY RODRIGUEZ. Email:
BIOCELL 2023, 47(5), 1135-1143. https://doi.org/10.32604/biocell.2023.027628
Received 07 November 2022; Accepted 16 January 2023; Issue published 10 April 2023
Abstract
Background: Capacitation is a set of physiological changes sperms undergo to acquire fertilizing capacity. In vivo, this process is directly associated with high calcium levels in sperm cytoplasm. Calcitriol, the vitamin D hypercalcemic metabolite, is related to human sperm motility, capacitation, and acrosome reaction. This work aimed to study the effect of calcitriol on bull sperm quality parameters and capacitation. Methods: One million freeze-thawed spermatozoa were obtained from different bulls and treated with 20 nM of calcitriol for 30 min. Untreated cells (negative control) and treated ones with calcitriol or heparin (100 µg/mL, positive capacitation control) were evaluated for motility, viability, and functional parameters. Menadione (70 µM, 30 min) treatment was included as a reactive oxygen species (ROS) positive sperm agent. Results: The results elucidated that sperm exposed to 20 nM calcitriol showed higher viability, vigor, and capacitation than their positive and negative controls. The percentage of sperm with intact plasma and acrosome membranes, mitochondrial membrane potential (ΔΨm), and phosphatidylserine externalization was similar in all the conditions evaluated, while ROS production was higher with heparin and menadione-treated groups than the calcitriol group or negative control. Conclusion: Our results indicate that calcitriol induces the capacitation of thawed bull spermatozoa and maintains acceptable values of progressive motility, viability, and vigor without altering key biological parameters such as redox status, ΔΨm, and cell death.Keywords
Sperm capacitation is a complex and indispensable physiological process that spermatozoa must undergo to acquire fertilizing ability (Molina et al., 2018). The principal cellular changes are: modification in plasma membrane cholesterol flux, increase in cell membrane permeability to bicarbonate and calcium ions (Ca2+), changes in proteins phosphorylation and protein kinase activity, and elevation of cyclic adenosine monophosphate (cAMP) concentration and intracellular pH (Ickowicz et al., 2012).
Uncapacitated spermatozoa maintain an acidic intracellular pH which acts as a negative regulator of calcium influx, preventing capacitation and the acrosome reaction (Vadnais et al., 2007). The role of calcium in the sperm is essential for motility, capacitation, acrosome reaction, as well as sperm chemotaxis (Beigi Harchegani et al., 2019). Finally, these changes in Ca2+ levels lead to modifications in the movement pattern of the sperm and its membrane composition to acquire the ability to fuse with the oocyte (Georgadaki et al., 2016).
Different media additives, both of animal and synthetic origin, have been used successfully to stimulate in vitro mammalian sperm capacitation and improve fertilization index. In this sense, several hormones present at the time of fertilization in the uterine and oviductal fluid may be good candidates to trigger sperm capacitation (Adeoya-Osiguwa et al., 2003; Therien, 2003).
Calcitriol or 1,25(OH)2D3, the active metabolite of vitamin D3, is a fat-soluble hormone widely known for its role in enhancing the intestinal absorption of calcium and phosphorus (Areco et al., 2020). Calcitriol elicits many of its pleiotropic effects through classical ligand-receptor signaling and downstream modulation of gene expression or indeed, through non-canonical genomic-independent mechanisms (Keane et al., 2017). Vitamin D receptor and calcitriol metabolizing enzymes such as 25-hydroxylase, 1α-hydroxylase, and 24-hydroxylase have been identified in testis and spermatozoa of humans (de Angelis et al., 2017) and domestic animals (Zhou et al., 2019). In addition, studies in humans showed that low concentrations of calcitriol induce sperm capacitation, due to which cholesterol flux and protein phosphorylation are stimulated, and sperm survival is increased (Aquila et al., 2008).
In recent years, it has been shown that different chemical natures of reactive oxygen species (ROS; superoxide anion, hydrogen peroxide, nitroxide) promote human sperm capacitation (Bui et al., 2018). The role of ROS in capacitation is dose-dependent. Low levels of ROS are necessary as key regulators to promote cholesterol flux, cAMP production, and tyrosine phosphorylation. On the other hand, excessive concentrations of ROS are toxic to sperm because they alter the composition and fluidity of sperm plasma membranes. Also, high levels of ROS change mitochondrial membrane potential, damage the genetic material, and activate cell death pathways which cause a failure in embryonic development (Alahmar, 2019).
Based on these previous observations, the aim of the current study was to determine the effect of calcitriol on bull sperm capacitation and sperm physiological parameters. Our data indicate that calcitriol induces capacitation in frozen-thawed bull sperm within a short period of time and improves cell viability and vigor, through a process associated with low ROS generation.
The experimental design is summarized in Fig. 1. Briefly, frozen straws (0.5 mL) from three different bulls were thawed in a water bath at 37°C for 30 s (n:3 per trial for control, heparin, and calcitriol treatments) and washed in sperm Tyrode’s albumin lactate pyruvate (TALP) medium (Sp-TALP). Then, 1 × 106 spermatozoa were incubated with 20 nM of calcitriol (Roche, Mannheim, Germany) for 30 min. Cells with 100 µg/mL heparin (Sigma-Aldrich, St. Louis, Missouri, USA) were included as a positive capacitation group in all assays. Sperm without treatment were used as a negative control. Subsequently, biological parameters, including: motility, vigor, ΔΨm, viability, plasma and acrosome membrane integrity, cell capacitation, phosphatidylserine (PS) externalization, ROS levels, and DNA integrity, were determined. All experiments were performed in triplicate, repeated at least three times, and data are presented as the mean ± SEM.
Figure 1: A flowchart of the experimental design.
Bovine semen straws were donated by the Artificial Insemination Center (CIAVT, Venado Tuerto, Santa Fé, Argentina). For the development of the experimental design, semen from three different bulls was used. The straws were thawed in a water bath at 37°C ± 2°C for 30 s and then resuspended in 1 mL of Sp-TALP (Parrish et al., 1988) with the addition of 6 mg/mL of bovine serum albumin (Sigma-Aldrich, St. Louis, Missouri, USA). To remove most of the cryoprotective diluent, samples were centrifuged twice at 300 × g for 5 min. One million spermatozoa were added and treated in each condition (control, calcitriol, and heparin).
Motility was evaluated by direct observation in a phase contrast microscope as described by Bosch et al. (2001). Briefly, 5–10 μL of bovine semen was placed on a prewarmed (37°C ± 2°C) microscope slide and examined at 200X magnification by phase contrast microscopy. The same trained technician evaluated the percentage of motile sperm throughout the study. Progressive sperm motility percentage was performed by counting a minimum of 100 sperm in at least five fields. The vigor of each of the treated and untreated samples was evaluated under the same conditions used for the progressive motility analysis. Sperm were scored according to a scale from 0 to 5, with 0 being non-motile sperm and 5% > 95% motility sperm with straight movement (Oliveira et al., 2015).
After each treatment, the percentage of live spermatozoa was evaluated by vital staining with propidium iodide (PI, Sigma-Aldrich, St. Louis, Missouri, USA). For this, the spermatozoa (2 × 105) were suspended in 0.2 mL of phosphate buffered saline (PBS) with 5 µg/mL of PI for 15 min at 37°C ± 2°C in the dark. The percentage of sperm incorporating the dye into their nucleus and fluorescence intensity were determined by a Guava flow cytometer (Guava easyCyte System, Merck KGaA, Darmstadt, Germany) with excitation and emission settings of 484 and 500 nm, respectively. Results were analyzed using FlowJo-V10 software (Tree Star Inc., Ashland, OR, USA).
Mitochondrial membrane potential (ΔΨm)
After treatments, the sperm cells (2 × 105) were resuspended and analyzed as described before in the viability assay. ΔΨm was assessed by cell staining with Rhodamine 123 (R123, Sigma-Aldrich, St. Louis, Missouri, USA). Cells were incubated with 10 µg/mL R123 in the dark for 15 min at 3°C. The mean fluorescence intensity of R123 was analyzed by flow cytometry with excitation and emission settings of 511 and 515–575 nm, respectively (Troiano et al., 1998).
Spermatozoa capacitation was determined as described by Collin et al. (2000). The chlortetracycline (CTC) stock solution containing 750 µM CTC-HCl, 130 mM NaCl, 5 mM L-cysteine, and 20 mM Tris acid (pH 7.8, Sigma-Aldrich, St. Louis, Missouri, USA) was prepared. 100 µL of sperm suspension was mixed with 150 µL of CTC solution in a tube at room temperature. Then, 3 mL of glutaraldehyde (12.5%) in a Tris base (2.5 M) was added as a fixative. The samples were covered with coverslips and observed using a microscope under blue-violet illumination (excitation at 400–440 nm and emission at 470 nm). Sperm cells were classified according to three different CTC staining patterns: whole head fluorescence (pre-capacitated cells), the fluorescence-free band in the post-acrosomal region (capacitated cells), and low fluorescence in sperm head except for a thin bright fluorescent band throughout the equatorial segment (acrosome-reacted cells). Sperm capacitation was determined by counting at least 200 spermatozoa per treatment.
To perform the hypoosmotic swelling test (HOST), 10 μL of semen from each condition was incubated in 500 μL of 0.45% NaCl for 30 min at 37°C ± 2°C. The samples were analyzed by phase contrast microscopy. Two hundred spermatozoa per treatment were quantified and classified according to the presence of coiled (intact plasma membrane) or uncoiled (damaged plasma membrane) tails (Jeyendran et al., 1984).
Phosphatidylserine externalization
PS externalization was performed according to the protocol recommended by the manufacturer and analyzed by flow cytometry. After different treatments, an aliquot of 1 × 105 sperm cells was incubated in the presence of 5 µL annexin V-FITC/250 µL in binding buffer for 15 min at 37°C (Thermo Fisher Scientific, Waltham, Massachusetts, USA). FITC detection was analyzed with a Guava flow cytometer with excitation and emission settings of 488 and 515 nm, respectively. Results were analyzed using the FlowJo-V10 software.
Reactive oxygen species levels
ROS production was evaluated by flow cytometry. After different treatments, sperm cells suspensions (5 × 105) were incubated with 100 µM 5-6-carboxy 2,7 dichlorodihydrofluorescein diacetate (H2DCFH-DA, Sigma-Aldrich, St. Louis, Missouri, USA) in PBS at 37°C ± 2°C for 10 min. Conversion of H2DCFH to DCF by ROS was analyzed with a Guava flow cytometer with excitation and emission settings of 484 and 535 nm, respectively. Results were analyzed using the FlowJo-V10 software. A ROS-positive control was included in this assay (sperms were incubated with 70 µM Menadione for 30 min; Guthrie and Welch, 2012).
Nuclear morphology was evaluated by Hoechst 33258 staining (Sigma-Aldrich, St. Louis, Missouri, USA). Control, heparin, calcitriol, and menadione-treated sperm were incubated at room temperature for 15 min with 1 µg/mL Hoechst 33258, washed with PBS, and placed on a glass slide and covered with a coverslip. Images of Hoechst 33258 staining (filter range: excitation 360 ± 40 nm and fluorescence emission 460 ± 50 nm) were acquired using a fluorescence microscope with a Nikon digital camera (Gong et al., 2009).
Data were analyzed by ANOVA with INFOSTAT/L software (di Rienzo et al., 2018). Post-hoc comparisons were performed using the Bonferroni's test. The values are expressed as means ± SEM and a p < 0.05 was considered statistically significant.
Effects of calcitriol on viability, motility, and mitochondrial membrane potential of bull-thawed spermatozoa
Effects of different concentrations of calcitriol (0.2, 2, 20 nM) were assessed on bovine sperm samples to evaluate the effects of the compound on cell motility, viability, and functional plasma membrane integrity for 30 and 60 min. Sperm viability, progressive motility, and functional plasma membrane integrity were not modified by any concentrations tested in any of the evaluated times (data not shown). For the remaining experiments, we used 20 nM calcitriol for 30 min.
Forty-five percent of sperm treated with calcitriol had progressive motility that was scored 4 ± 0.1 for vigor. On the other hand, the cells that were exposed to 100 µg/mL heparin showed similar motility (42.5% ± 4.79%) but lower vigor (2.75% ± 0.25%; p ≤ 0.05; Table 1). To evaluate the possibility of a positive correlation between progressive motility, and functional integrity of mitochondria, ΔΨm was determined. The median fluorescence intensity of sperm was not modified with different treatments (control, heparin, or calcitriol; Table 1).
As shown in Fig. 2, sperm viability was evaluated by flow cytometry (PI exclusion assay). Cells treated with 20 nM calcitriol for 30 min presented a higher percentage of viable cells (40.1% ± 3.76%) than those treated with heparin (24.6% ± 0.2%; p ≤ 0.05).
Figure 2: Cell viability was determined from sperm samples stained with 5 µg/mL propidium iodide (PI) by flow cytometry. Values indicate means ± SEM from at least three independent replicates. The histograms are representative of three independent experiments. *p ≤ 0.05 vs. % heparin treatment.
Effects of calcitriol on plasma and acrosome membranes integrities and phosphatidylserine externalization of bull-thawed spermatozoa
Percentages of the hypoosmotic swelling test (HOST) positive cells and with PS externalization (Annexin V %) were similar among groups (control, heparin, calcitriol; Table 2). These results indicate that membrane functional integrity was not affected by the treatments. Also, acrosome integrity did not change within treatments (Table 2).
Effects of calcitriol on levels of reactive oxygen species and nuclear morphology of bull-thawed spermatozoa
ROS levels were studied in different treatments to evaluate the putative association of oxidative stress with capacitation triggered by heparin or calcitriol. As shown in Fig. 3A, ROS concentrations significantly increased with 70 µM menadione (positive control; Guthrie and Welch, 2012). Sperm treated with calcitriol had lower ROS concentration (67.87 ± 3.43%) compared to that in the heparin sperm group (102.77% ± 11.75%; p ≤ 0.05; Fig. 3B).
Figure 3: Effects of calcitriol or heparin on reactive oxygen species (ROS) production in bull sperm cells. Spermatozoa were treated with 20 nM calcitriol or 100 µg/mL heparin for 30 min. ROS production was analyzed by flow cytometry. (A) Histograms (one for each treatment) showing the distribution of events according to their fluorescence intensity. The selected graph is representative of all replicates (four independent replicates). (B) Mean fluorescence intensity of sperm from control, heparin, calcitriol, and menadione (70 µM, ROS positive control) groups. *p ≤ 0.05 vs. all conditions. **p ≤ 0.05 vs. ROS levels in the calcitriol group.
Nuclear morphology was studied to evaluate the effect of ROS on DNA integrity. As seen in Fig. 4, sperm treated with 70 µM menadione had a significant increase in altered nuclear morphology percentage compared to other sperm treatments.
Figure 4: Effects of calcitriol or heparin on nuclear morphology in bull sperm cells. (A) Fluorescent microphotographs showing sperm nuclear morphology. Arrows show bull spermatozoa with altered nuclear morphology; scale bars 10 μm. (B) Percentage of altered nuclear morphology of bovine spermatozoa treated with calcitriol, heparin, or menadione. Values indicate means ± SEM from at least three independent replicates. *p ≤ 0.05 vs. control group.
Effects of calcitriol on capacitation of bull-thawed spermatozoa
Fig. 5A depicts representative microphotographs of sperm displaying different CTC staining patterns. Incubation of spermatozoa with 20 nM calcitriol caused a significant increase in the percentage of capacitated sperm compared to those in the negative control and heparin (positive capacitation control) groups (p ≤ 0.05, Fig. 5B).
Figure 5: Capacitation of bovine spermatozoa treated with calcitriol or heparin. (A) Chlortetracycline (CTC) staining patterns for pre-capacitated, capacitated, and acrosome-reacted sperm. Scale bars 10 μm. (B) Percentages of capacitated sperm were determined by the epifluorescence CTC technique. Values indicate means ± SEM from at least three independent replicates. *p ≤ 0.05 vs. control and calcitriol group, **p ≤ 0.05 vs. heparin treatment.
The present data demonstrated that hypercalcemic metabolite of vitamin D, calcitriol, rapidly increases the capacitation and vigor of frozen-thawed bull sperm, maintaining acceptable values of progressive motility, ΔΨm, plasma and acrosome integrity, and nuclear morphology. The viability of sperm exposed to calcitriol was higher than those with heparin treatment, while ROS levels induced by calcitriol were similar to control cells and were lower than that observed in the heparin treatment group.
Capacitation is a physiological process whereby spermatozoa are prepared for engagement with the oocyte (Aitken and Nixon, 2013). Sperm capacitation includes multiple physiological modifications associated with hyperactivated motility, hyperpolarization of the plasma membrane, and an increase in intracellular pH, calcium, and cyclic adenosine monophosphate levels (Ickowicz et al., 2012). Also, a recent work demonstrated a direct relationship between the mitochondrial membrane potential and the capacitation of rat spermatozoa (Giaccagli et al., 2021).
The sperm capacitation process was also influenced by frozen-thawing. Cryo-capacitation of human and domestic animals was associated with plasma membrane reorganization (Bailey et al., 2000; Benko et al., 2022). Cryopreservation also has deleterious effects on spermatozoa, especially on plasmalemma, acrosomes, and tails (Ozkavukcu et al., 2008). Different biochemical processes such as lipid and protein oxidation, decreased motility, viability, mitochondrial injury, and sperm DNA fragmentation can be observed in sperms of human and farm animals pos-thawing, leading to a decline in the quality of freeze-thawed cells (Kumar et al., 2016; Khan et al., 2021).
Heparin is a well-known agent that induces bull sperm capacitation. Parrish (Parrish et al., 1988; Parrish et al., 1989; Parrish, 2014) described that heparin activates the capacitation mechanism in fresh bull spermatozoa. These studies demonstrated that capacitation of bovine sperm by heparin requires at least a 4 h incubation time. In our study, the cryopreserved sperm treated with heparin for 30 min increased sperm capacitation (38.87% ± 2.51%, p ≤ 0.05 vs. Control 25.17% ± 1.77%). Similar results were demonstrated using a selective gradient method prior to heparin treatment (Aguiar et al., 2019).
Heparin primarily acts on the sperm membrane (Parrish, 2014), where it facilitates the entry of ions, especially calcium. Calcium intake leads to an increase in the intracellular pH and, consequently, hyperactivation (Gangwar and Atreja, 2015). The increase in intracellular calcium concentrations, in turn, activates the soluble adenylate cyclase (sAC), stimulating the cAMP synthesis and protein tyrosine phosphorylation through protein kinase A (PKA). In the same line, Cormier and Bailey (Cormier and Bailey, 2003) showed that two phosphotyrosine-containing proteins (56-PP and 114-PP) seemed to be modulated by heparin when freshly extended spermatozoa were incubated for up to 5 h, but only 56-PP was already evident in cryopreserved spermatozoa.
This work describes for the first time the use of calcitriol for bovine sperm capacitation. Bull sperm from thawed straws treated with 20 nM calcitriol for 30 min showed an increased percentage of capacitated cells and viability than heparin treatment. It was recently shown that the supplementation of semen extender with different concentrations of vitamin D increases the motility and vigor of bulls with normozoospermia and does not alter the acrosome integrity rate (Asadpour et al., 2021). In this line, Zanatta et al. (2017) reported that human sperm incubated in calcitriol-containing media increased cholesterol efflux, protein phosphorylation, and sperm capacitation and survival. The nongenomic actions of calcitriol occur within 1–45 min through a mechanism that involves calcium interaction with intracellular signaling pathways (de Angelis et al., 2017). Voltage-gated calcium channels like CatSper 1 and 2, calcium ATPase inactivation, and the sodium-calcium pump, have been postulated to result in a calcium influx. An increase in intracellular concentrations of calcium plays an important role in the process of capacitation since it activates sAC/cAMP/PKA protein phosphorylation and membrane hyperpolarization (Vadnais et al., 2007).
Sperm oxidative stress has been associated with damage to the sperm plasma membrane and chromatin as well as alterations in signal transduction mechanisms, which in turn can impair fertility (Du Plessis et al., 2010). To analyze these consequences, we evaluated the integrity of sperm plasma and acrosome membranes. Calcitriol and heparin treatments altered neither sperm plasma membrane nor acrosome integrity. Furthermore, the evaluation of apoptotic early cell death by analysis of PS externalization from the inner to the outer leaflet of the plasma membrane showed that calcitriol treatment did not modify this parameter compared to heparin treatment and control groups. DNA fragmentation index is another parameter correlated with alterations in sperm morphology and with an excessive amount of ROS (Cervantes et al., 2019). Our results showed that menadione treatment (positive control of ROS generation) induced high levels of ROS and nuclear morphological alterations. No alterations were observed in nuclear morphology when bovine sperm were treated with calcitriol and heparin for 30 min.
Although more studies are necessary to elucidate the molecular mechanisms that are involved in the capacitation-inducing effect caused by calcitriol, the relevance of the results obtained in this work can be directly applied to both in vitro and in vitro in reproductive biology assays.
We report for the first time the use of vitamin D active metabolite calcitriol as a sperm capacitation inducer agent for the bovine species. A significant population of thawed bull spermatozoa was capacitated with calcitriol in a brief period. The achieved values are higher than those observed after heparin treatment. Calcitriol treatment improved spermatozoa viability and vigor and maintained plasma and acrosome membrane integrity. Unlike heparin and menadione exposure, calcitriol maintains sperm ROS levels similar to those in non-treated sperm (control). Calcitriol treatment, as demonstrated here, proves to be an alternative approach to achieve sperm capacitation of freeze-thawed bull spermatozoa for in vitro fertilization in cattle.
Acknowledgement: We are grateful to Centro de Inseminación Artificial de Venado Tuerto (CIAVT) for providing the frozen semen.
Funding Statement: Research was funded by grants from National University of Río Cuarto (UNRC) through the Secretary of Science and Technology (SECYT, PPI 2020-2022, Res 083).
Author Contributions: All authors contributed to the study conception and design. Material preparation, data collection, and analysis were performed by Liaudat Ana Cecilia. The first draft of the manuscript was written by Liaudat Ana Cecilia and Bosch Pablo, and all authors commented on previous versions of the manuscript. All authors have read and approved the final manuscript.
Availability of Data and Materials: The datasets generated during the current study are available from the corresponding author upon reasonable request.
Ethics Approval: Not applicable.
Conflicts of Interest: The authors declare that they have no conflicts of interest to report regarding the present study.
References
Adeoya-Osiguwa SA, Markoulaki S, Pocock V, Milligan SR, Fraser LR (2003). 17β-Estradiol and environmental estrogens significantly affect mammalian sperm function. Human Reproduction 18: 100–107. https://doi.org/10.1093/humrep/deg037 [Google Scholar] [PubMed] [CrossRef]
Aguiar GB, Caldas-Bussiere MC, Maciel VL, de Carvalho CSP, de Souza CLM (2019). Association of L-arginine with heparin on the sperm capacitation improves in vitro embryo production in bovine. Animal Reproduction 16: 938–944. https://doi.org/10.21451/1984-3143-AR2019-0022 [Google Scholar] [PubMed] [CrossRef]
Aitken RJ, Nixon B (2013). Sperm capacitation: A distant landscape glimpsed but unexplored. Molecular Human Reproduction 19: 785–793. https://doi.org/10.1093/molehr/gat067 [Google Scholar] [PubMed] [CrossRef]
Alahmar A (2019). Role of oxidative stress in male infertility: An updated review. Journal of Human Reproductive Sciences 12: 4–18. https://doi.org/10.4103/jhrs.JHRS_150_18 [Google Scholar] [PubMed] [CrossRef]
Aquila S, Guido C, Perrotta I, Tripepi S, Nastro A, Andò S (2008). Human sperm anatomy: Ultrastructural localization of 1α,25-dihydroxyvitamin D3 receptor and its possible role in the human male gamete. Journal of Anatomy 213: 555–564. https://doi.org/10.1111/j.1469-7580.2008.00975.x [Google Scholar] [PubMed] [CrossRef]
Areco VA, Kohan R, Talamoni G, Tolosa de Talamoni NG, Peralta López ME (2020). Intestinal Ca2+ absorption revisited: A molecular and clinical approach. World Journal of Gastroenterology 26: 3344–3364. https://doi.org/10.3748/wjg.v26.i24.3344 [Google Scholar] [PubMed] [CrossRef]
Asadpour R, Taravat M, Rahbar M, Khoshniyat M, Hamidian G. (2021). Effects of vitamin D supplementation in extender on sperm kinematics and apoptosis following the freeze-thaw process in normozoospermic and asthenozoospermic Holstein bulls. Basic and Clinical Andrology 31: 20. https://doi.org/10.1186/s12610-021-00137-5 [Google Scholar] [PubMed] [CrossRef]
Bailey JL, Bilodeau JF, Cormier N (2000). Minireview: Semen cryopreservation in domestic animals: A damaging and capacitating phenomenon. Journal of Andrology 21: 1–7. https://doi.org/10.1002/j.1939-4640.2000.tb03268.x [Google Scholar] [CrossRef]
Beigi Harchegani A, Irandoost A, Mirnamniha M, Rahmani H, Tahmasbpour E, Shahriary A (2019). Possible mechanisms for the effects of calcium deficiency on male infertility. International Journal of Fertility and Sterility 12: 267–272. https://doi.org/10.22074/ijfs.2019.5420 [Google Scholar] [PubMed] [CrossRef]
Benko F, Ďuračka M, Baňas Š., Lukáč N, Tvrdá E (2022). Biological relevance of free radicals in the process of physiological capacitation and cryocapacitation. Oxygen 2: 164–176. https://doi.org/10.3390/oxygen2020014 [Google Scholar] [CrossRef]
Bosch P, de Avila JM, Ellington JE, Wright RW (2001). Heparin and Ca2+-free medium can enhance release of bull sperm attached to oviductal epithelial cell monolayers. Theriogenology 56: 247–260. https://doi.org/10.1016/S0093-691X(01)00560-X [Google Scholar] [PubMed] [CrossRef]
Bui AD, Sharma R, Henkel R, Agarwal A (2018). Reactive oxygen species impact on sperm DNA and its role in male infertility. Andrologia 50: e13012. https://doi.org/10.1111/and.13012 [Google Scholar] [PubMed] [CrossRef]
Cervantes IE, Durán MLA, Carballo ME, et al. (2019). Sperm capacitation, a tool for assisted reproduction techniques. Acta Medica 17: 34–41. [Google Scholar]
Collin S, Sirard MA, Dufour M, Bailey JL (2000). Sperm calcium levels and chlortetracycline fluorescence patterns are related to the in vivo fertility of cryopreserved bovine semen. Journal of Andrology 21: 938–943. https://doi.org/10.1002/j.1939-4640.2000.tb03425.x [Google Scholar] [CrossRef]
Cormier N, Bailey JL (2003). A differential mechanism is involved during heparin- and cryopreservation-induced capacitation of bovine spermatozoa. Biology of Reproduction 69: 177–185. https://doi.org/10.1095/biolreprod.102.011056 [Google Scholar] [PubMed] [CrossRef]
de Angelis C, Galdiero M, Pivonello C, Garifalos F, Menafra D et al. (2017). The role of vitamin D in male fertility: A focus on the testis. Reviews in Endocrine and Metabolic Disorders 18: 285–305. https://doi.org/10.1007/s11154-017-9425-0 [Google Scholar] [PubMed] [CrossRef]
di Rienzo JA, Casanoves F, Balzarini MG, Gonzalez L, Tablada M, Robledo CW (2018). InfoStat versión 2018. Grupo InfoStat, FCA, Universidad Nacional de Córdoba, Argentina. http://www.infostat.com.ar [Google Scholar]
Du Plessis SS, McAllister DA, Luu A, Savia J, Agarwal A, Lampiao F (2010). Effects of H2O2 exposure on human sperm motility parameters, reactive oxygen species levels and nitric oxide levels. Andrologia 42: 206–210. https://doi.org/10.1111/(ISSN)1439-0272 [Google Scholar] [CrossRef]
Gangwar DK, Atreja SK (2015). Signalling events and associated pathways related to the mammalian sperm capacitation. Reproduction in Domestic Animals 50: 705–711. https://doi.org/10.1111/rda.12541 [Google Scholar] [PubMed] [CrossRef]
Georgadaki K, Khoury N, Spandidos DA, Zoumpourlis V (2016). The molecular basis of fertilization (Review). International Journal of Molecular Medicine 38: 979–986. https://doi.org/10.3892/ijmm.2016.2723 [Google Scholar] [PubMed] [CrossRef]
Giaccagli MM, Gómez-Elías MD, Herzfeld JD, Marín-Briggiler CI, Cuasnicú PS, Cohen DJ, da Ros VG (2021). Capacitation-induced mitochondrial activity is required for sperm fertilizing ability in mice by modulating hyperactivation. Frontiers in Cell and Developmental Biology 9: 1–13. https://doi.org/10.3389/fcell.2021.767161 [Google Scholar] [PubMed] [CrossRef]
Gong Y, Wu J, Huang Y, Shen S, Han X (2009). Nonylphenol induces apoptosis in rat testicular Sertoli cells via endoplasmic reticulum stress. Toxicology Letters 186: 84–95. https://doi.org/10.1016/j.toxlet.2009.01.010 [Google Scholar] [PubMed] [CrossRef]
Guthrie HD, Welch GR (2012). Effects of reactive oxygen species on sperm function. Theriogenology 78: 1700–1708. https://doi.org/10.1016/j.theriogenology.2012.05.002 [Google Scholar] [PubMed] [CrossRef]
Ickowicz D, Finkelstein M, Breitbart H (2012). Mechanism of sperm capacitation and the acrosome reaction: Role of protein kinases. Asian Journal of Andrology 14: 816–821. https://doi.org/10.1038/aja.2012.81 [Google Scholar] [PubMed] [CrossRef]
Jeyendran RS, van der Ven HH, Perez-Pelaez M, Crabo BG, Zaneveld LJ (1984). Development of an assay to assess the functional integrity of the human sperm membrane and its relationship to other semen characteristics. Journal of Reproduction and Fertility 70: 219–228. https://doi.org/10.1530/jrf.0.0700219 [Google Scholar] [PubMed] [CrossRef]
Keane KN, Cruzat VF, Calton EK, Hart PH, Soares MJ, Newsholme P, Yovich JL (2017). Molecular actions of vitamin D in reproductive cell biology. Reproduction 153: R29–R42. https://doi.org/10.1530/REP-16-0386 [Google Scholar] [PubMed] [CrossRef]
Khan IM, Cao Z, Liu H, Khan A, Rahman SU, Khan MZ, Sathanawongs A, Zhang Y (2021). Impact of cryopreservation on spermatozoa freeze-thawed traits and relevance OMICS to assess sperm cryo-tolerance in farm animals. Frontiers in Veterinary Science 8: 1–14. https://doi.org/10.3389/fvets.2021.609180 [Google Scholar] [PubMed] [CrossRef]
Kumar D, Kumar P, Singh P, Yadav SP, Yadav PS (2016). Assessment of sperm damages during different stages of cryopreservation in water buffalo by fluorescent probes. Cytotechnology 68: 451–458. https://doi.org/10.1007/s10616-014-9798-9 [Google Scholar] [PubMed] [CrossRef]
Molina LCP, Luque GM, Balestrini PA, Marín-Briggiler CI, Romarowski A, Buffone MG (2018). Molecular basis of human sperm capacitation. Frontiers in Cell and Developmental Biology 6: 72. https://doi.org/10.3389/fcell.2018.00072 [Google Scholar] [PubMed] [CrossRef]
Oliveira KG, Leão DL, Almeida DVC, Santos RR, Domingues SFS (2015). Seminal characteristics and cryopreservation of sperm from the squirrel monkey, Saimiri collinsi. Theriogenology 84: 743–749.e1. https://doi.org/10.1016/j.theriogenology.2015.04.031 [Google Scholar] [PubMed] [CrossRef]
Ozkavukcu S, Erdemli E, Isik A, Oztuna D, Karahuseyinoglu S (2008). Effects of cryopreservation on sperm parameters and ultrastructural morphology of human spermatozoa. Journal of Assisted Reproduction and Genetics 25: 403–411. https://doi.org/10.1007/s10815-008-9232-3 [Google Scholar] [PubMed] [CrossRef]
Parrish JJ (2014). Bovine in vitro fertilization: In vitro oocyte maturation and sperm capacitation with heparin. Theriogenology 81: 67–73. https://doi.org/10.1016/j.theriogenology.2013.08.005 [Google Scholar] [PubMed] [CrossRef]
Parrish JJ, Susko-Parrish JL, First NL (1989). Capacitation of bovine sperm by heparin: Inhibitory effect of glucose and role of intracellular pH. Biology of Reproduction 41: 683–699. https://doi.org/10.1095/biolreprod41.4.683 [Google Scholar] [PubMed] [CrossRef]
Parrish JJ, Susko-Parrish J, Winer MA, First NL (1988). Capacitation of bovine sperm by heparin. Biology of Reproduction 38: 1171–1180. https://doi.org/10.1095/biolreprod38.5.1171 [Google Scholar] [PubMed] [CrossRef]
Therien I (2003). Effect of progesterone on bovine sperm capacitation and acrosome reaction. Biology of Reproduction 69: 1408–1415. https://doi.org/10.1095/biolreprod.103.017855 [Google Scholar] [PubMed] [CrossRef]
Troiano L, Granata ARM, Cossarizza A, Kalashnikova G, Bianchi R, Pini G, Tropea F, Carani C, Franceschi C (1998). Mitochondrial membrane potential and DNA stainability in human sperm cells: A flow cytometry analysis with implications for male infertility. Experimental Cell Research 241: 384–393. https://doi.org/10.1006/excr.1998.4064 [Google Scholar] [PubMed] [CrossRef]
Vadnais ML, Galantino-Homer HL, Althouse GC (2007). Current concepts of molecular events during bovine and porcine spermatozoa capacitation. Archives of Andrology 53: 109–123. https://doi.org/10.1080/01485010701329386 [Google Scholar] [PubMed] [CrossRef]
Zanatta AP, Brouard V, Gautier C, Goncalves R, Bouraïma-Lelong H, Mena Barreto Silva FR, Delalande C (2017). Interactions between oestrogen and 1α,25(OH)2-vitamin D3 signalling and their roles in spermatogenesis and spermatozoa functions. Basic and Clinical Andrology 27: 1–14. https://doi.org/10.1186/s12610-017-0053-z [Google Scholar] [PubMed] [CrossRef]
Zhou P, McEvoy TG, Gill AC, Lambe NR, Morgan-Davies CR, Hurst E, Sargison ND, Mellanby RJ (2019). Investigation of relationship between vitamin D status and reproductive fitness in Scottish hill sheep. Scientific Reports 9: 1–10. https://doi.org/10.1038/s41598-018-37843-6 [Google Scholar] [PubMed] [CrossRef]
Cite This Article
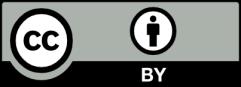
This work is licensed under a Creative Commons Attribution 4.0 International License , which permits unrestricted use, distribution, and reproduction in any medium, provided the original work is properly cited.