Open Access
REVIEW
Fe-dependent cellular alterations of oxidative balance in aquatic organisms. Could be ferroptosis involved?
1 Fisicoquímica, Facultad de Farmacia y Bioquímica, Universidad de Buenos Aires, Buenos Aires, C1113 AAD, Argentina
2 Instituto de Bioquímica y Medicina Molecular (IBIMOL), CONICET-Universidad de Buenos Aires, Buenos Aires, C1113 AAD, Argentina
* Corresponding Author: SUSANA PUNTARULO. Email:
BIOCELL 2023, 47(5), 1177-1189. https://doi.org/10.32604/biocell.2023.027107
Received 18 October 2022; Accepted 26 December 2022; Issue published 10 April 2023
Abstract
The purpose of this review is to briefly summarize the central role of iron (Fe) in terms of cellular alterations of the oxidative/protective balance with special emphasis on its possible involvement in ferroptosis-dependent disruption in aquatic organisms. In ferroptotic cells or tissues, the intracellular Fe level increases; meanwhile the treatment with Fe chelators limits ferroptosis. Eukaryotic algae can assimilate Fe from the environment through several mechanisms, and aquatic animals incorporate dissolved Fe and Fe bound to both inorganic particles and organic matter. The central role of lipid peroxidation mediating ferroptosis was demonstrated in some algae where both low and high Fe concentrations could induce oxidative stress and programmed cell death. Aquatic animals have high levels of polyunsaturated fatty acids and numerous studies have analyzed Fe effects on the lipidic fraction which could be related to ferroptosis. The ferroptosis reaction can be regulated through the antioxidant defense system, in combination with the protein degradation structure, metabolism, and gene transcription. Early depletion of non-enzymatic antioxidants like reduced glutathione (GSH) in animals, and the reduction of both GSH and ascorbate in photosynthetic organisms, are characteristic features of ferroptosis. Therefore, ferroptosis can be prevented if Fe chelators, certain antioxidants, and specifically regulating genes are activated. Thus, the global scenario for the Fe role as a toxic component in biological systems seems to be even more complicated than it was previously understood. Much more research on this subject is needed to improve the life span and survival of aquatic organisms after exposure to natural and anthropogenic adverse conditions.
Keywords
Iron (Fe) is an essential element for the growth and life maintenance of heterotrophic and autotrophic aquatic organisms (González et al., 2012a). Fe can be naturally abundant in certain regions or could be incorporated into water bodies by human actions. Eolian deposition of dust, river discharge, washout of particles in the atmosphere by rainfall, groundwater discharge, glacial melting, volcanic sediments, coastal erosion, and upwelling of Fe-rich deep waters over hydrothermal vents are among the natural Fe sources (Watson, 2001). Meanwhile, nanoparticles, chemical and mining industries, disposal of waste metal, ports, and eolian deposition of atmospheric dust from polluted areas are a few of such anthropogenic factors. Final Fe concentration in seawater depends on the area under consideration. The environmental conditions may act, adding potential stress to the aquatic biota since sediments can act either as a major reservoir (Caccia et al., 2003) or as a source of Fe (Adams et al., 1992) and other chemical elements.
Fe could act as a potent toxicant which has a wide spectrum of adverse effects generating adverse consequences in aquatic organisms. At the cellular physiological pH (7.0), both ferric (Fe3+) and ferrous (Fe2+) forms are present as complexes with low molecular weight compounds reported as the labile Fe pool (LIP). A permanent Fe flux from the extracellular medium to the cytoplasm is generated (González et al., 2010). Terms such as “redox-active” or “chelatable Fe” have been also used when referring to LIP (Koppenol and Hider, 2019). Different ligands have been associated with cytosolic LIP. Glutathione (GSH), a molecule present in eukaryotic cells at relatively high concentrations, is considered a key Fe2+ ligand forming a 1:1 complex (Hider and Kong, 2011). Hider and Kong (2013) proposed that GSH is the major chelator to form “low molecular weight” Fe complexes in mitochondria. Fe concentration was estimated to be much higher than 1 μM inside the lysosome, due to the continued breakdown of ferritin (Ft) (Kurz et al., 2007). The LIP is well known as an oxidative stress inducer through the increase in cellular levels of reactive oxygen species (ROS). The one-electron reduction of hydrogen peroxide (H2O2) by a Fe2+ complex in the Fenton reaction is an important contribution to the biological environment (Koppenol and Bounds, 2011). Fe can be involved in the initiation and propagation of free radical generation (Lushchak, 2011). These reactive species could trigger damage at the lipidic, DNA, and protein levels (Regoli et al., 2004), enzyme inhibition, weakening of cell signaling, disruption of calcium homeostasis, and modifications in gene regulation (Stohs and Bagchi, 1995).
Xenobiotic uptake by phytoplankton is the first step in bioaccumulation processes in aquatic food webs. The water quality can depend on the type and number of algal species. Estévez et al. (2001) demonstrated the induction of oxidative stress due to an excess of Fe content in Chlorella vulgaris cultures. This response, coupled with other severe environmental conditions could affect cellular growth and have a negative biogeoimpact on phytoplankton. Fe uptake is strictly required for phytoplankton development since the photosynthetic complex contains numerous Fe loci. Ocean primary productivity may be limited by deficiency of this essential element in some regions (Martin and Fitzwater, 1988). On the other hand, Fe can control plankton blooms, which also affects the biogeochemical cycles of C, N, Si, and S, influencing the climate system of the earth (Boyd et al., 2007). Zooplankton can aid in Fe mobilization by acidic digestion (Moore et al., 1984), and grazing is an essential factor in recycling biogenic Fe, making it highly bioavailable (Hutchins et al., 1999; Nuester et al., 2014). The mobilized Fe can be released in a dissolved manner directly from krill, or via multiple pathways involving microbes, other zooplankton species, and krill predators. Then, substantial amounts of bioavailable Fe can be delivered to the water column contributing to the fertilization of coastal areas and the ocean itself. For example, Nuester et al. (2014) observed that diatoms take up 43- to 73-faster the regenerated Fe by copepods than the inorganic Fe. Copepods also release Fe-binding ligands when grazing on phytoplankton (Sato et al., 2007). The ligands can form complexes with inorganic Fe and, thereby, increase Fe solubility. Filters and deposit feeders ingest Fe bound to dissolved and particulate matter in the water column and the sediment surface, but also dissolved Fe can be absorbed into respiratory surfaces (González et al., 2015).
In recent years, a new form of Fe-dependent cell death, termed ferroptosis, was described in terrestrial animals and plants (Dixon et al., 2012). This regulated cell death usually accompanies a large amount of Fe accumulation and lipid peroxidation (LP) (Li et al., 2021). However, ferroptosis is poorly studied in aquatic environments and organisms. The purpose of this manuscript is to briefly summarize the central role of Fe in terms of cellular alterations of the oxidative/protective balance, with special emphasis on its possible involvement in ferroptosis disruption in aquatic organisms.
Fe and ferroptosis: General characteristics
Ferroptosis has been described as a complex mechanism involving (i) morphological, (ii) biochemical, (iii) genetic, and (iv) protein hallmarks (Chen et al., 2021). Consequently, this kind of programmed cell death could have a great impact on growth, tissue homeostasis, and the development of diverse pathological conditions and diseases. The proliferation of the inducing signal occurs upstream of cell rupture and involves the spread of a cell swelling effect through cell populations (Riegman et al., 2020). However, the main studies on this issue have been performed on vertebrates and have been focused on clinical consequences. The existence of these pathways in invertebrates and other aquatic organisms is still in the initial stages of being proved.
The biochemical pathways involved in ferroptosis, a Fe-dependent regulated cell death (RCD), are the initial aspects that are studied or interpreted in aquatic organisms. These pathways are related to Fe cellular accessibility and accumulation, LP, and the dysfunction or decrease of the antioxidant ability of the defense system. Fe accumulation could be a biochemical sign of ferroptosis, mediating biochemical events (e.g., elevated Fenton reaction or activated Fe-containing enzymes) (Chen et al., 2020). In ferroptotic cells or tissues, the intracellular or mitochondrial Fe2+ level is increased, and the treatment with Fe chelators, like deferoxamine, limits ferroptosis both, in vitro (Dixon et al., 2012) and in vivo (Lu et al., 2020).
Fe is allocated in several subcellular organelles (e.g., mitochondria and lysosomes). Fe concentration in cells is regulated by a complex network that entails the absorption, storage, utilization, and discharge of the metal (Chen et al., 2020). Specific molecular regulators, which are related to Fe homeostasis, control ferroptosis sensitivity. For example, Fe uptake facilitated by transferrin (TF; Gao et al., 2015), lactotransferrin (LTF; Wang et al., 2020), TF receptor (TFRC; Gao et al., 2015), and the nuclear receptor coactivator 4 (NCOA4)-dependent Ft degradation (Hou et al., 2016) promote ferroptotic cell death. According to Feng et al. (2020), TFRC is considered a biomarker of ferroptosis in cell cultures or tissues. Consequently, the presence of the anti-TFRC antibody (called 3F3-FMA) indicates ferroptotic cell death or damage. Studies of this nature have not yet been performed either in invertebrates or in photosynthetic aquatic organisms.
Eukaryotic algae can assimilate Fe from the environment through several mechanisms: release of siderophores, which are high affinity Fe-binding molecules (Krachler et al., 2019), Fe3+ reduction by membrane ferric reductases, assimilation of photochemically reduced Fe3+ or Fe3+–L (L = low affinity Fe-binding molecules; Shaked et al., 2005), or by assimilation of bacteria and particulate minerals (Nodwell and Price, 2001). Uptake and use of Fe in metabolic activities by microalgae require a water-soluble form of Fe (Fe2+) (Juneja et al., 2013). The presence of the Fe2+ form is needed in phytoplankton biomass growth and may facilitate the enhanced production of energy molecules, like lipids (Liu et al., 2008; Ghafari et al., 2018; Rana et al., 2020). However, under Fe-limited circumstances, the rate of light absorption and light utilization in the electron transport system is imbalanced. For example, Fan et al. (2014) reported a 22.1% decrease in chlorophyll concentration under Fe-deficient conditions. Nevertheless, high Fe concentrations also have negative responses in microalgal cells decreasing their growth rate (Fan et al., 2014).
During food ingestion, aquatic animals incorporate dissolved Fe and Fe bound to both inorganic particles and organic matter. In lobsters, Fe2+ is absorbed by the cells through a divalent cation exchanger (Chavez-Crooker et al., 2001). In the digestive glands of the bivalve Mya arenaria the concentration of Fe3+ appears to be significantly lower than the concentration of Fe2+ in the LIP (González et al., 2012b). Another study observed that the LIP represented 10% of the total Fe content in the digestive gland of the same bivalve species (González et al., 2010), meanwhile, the LIP denoted a 3.5% of the total Fe content in the digestive gland of the bivalve Laternula elliptica (González and Puntarulo, 2011). Thus, a relatively more active Fe sequestration process can be suggested as a part of the safe cell compartments in L. elliptica than in M. arenaria. This information could indicate that L. elliptica has a better adaptation to an ecosystem with higher Fe natural marine content than M. arenaria.
Cellular injury and toxic effects related to Fe
Fe content in aquatic ecosystems could be a main stressor with a major role in LP in photosynthetic unicellular species and multicellular heterotrophic organisms, such as invertebrates and vertebrates (González et al., 2012c). LP is defined as the oxidative damage of lipids with two or more instaurations (Hummel et al., 2006). Polyunsaturated fatty acids (PUFA) are critical targets for powerful oxidizing species such as ROS. This mechanism of damage produces lipid hydroperoxides, one of the most significant hallmarks of ferroptosis (Dixon and Stockwell, 2014; Kuang et al., 2020). Therefore, LP is widely accepted to play a key role in mediating ferroptosis (Yang and Stockwell, 2016).
Thamatrakoln et al. (2012) and Luo et al. (2014) demonstrated that in some microalgae, such as Thalassiosira pseudonana diatoms, both low and high Fe concentrations can lead to oxidative stress and the induction of RCD. Estévez and Puntarulo (2005) reported that at high Fe concentrations (500 µM Fe), oxidative damage was involved in the deleterious effects seen in C. vulgaris. However, at moderate concentrations (50 µM Fe), the metal administration not only improved the nutritional state but also increased the metabolic activity of the microalgae. C. vulgaris cells showed an increase of lipid radical (LR•) EPR signals in a Fe dose-dependent manner (Estévez et al., 2001). Quantification of LR• in algal supplemented with Fe exhibited a significantly increased in the LR• content in the membranes at a higher tested dose of Fe (500 µM), as compared to those supplemented with 5 and 90 µM Fe. Robello et al. (2021) evaluated the supplementation of Fe at the latent growth phase in the marine diatom Fragilaria sp. In the presence of 50 µM Fe, the cells showed a significant reduction in the hydrophilic and lipophilic redox balance, as compared to control values. Significant changes in the LR• content, generated as a result of growth or Fe supplementation with 0.3 and 50 µM Fe, were also observed. González et al. (2017) quantified the LR•-dependent EPR signals in two Antarctic macroalgae, Gigartina skottsbergii and Himantothallus grandifolius, from two different areas. They found that, in both species, LR• content was significantly higher in the macroalgae inhabiting the Island D zone, heavily influenced by a glacier sediment inflow with high Fe input, as compared to samples isolated from specimens collected in Peñón de Pesca, a zone with low Fe presence. Even more, both algae showed changes in the lipophilic redox balance in environments with different Fe inputs (González et al., 2017).
Other stressors, like temperature, could also stimulate LP-related ferroptosis. Aguilera et al. (2021) measured in the cyanobacteria Synechocystis sp. cytosolic ROS and LP accumulation after heat shock at 50°C heat. While the major level of cytosolic ROS was detected 1 h after heat shock, the accumulation of lipid peroxides reached a maximum of 3 h after the treatment. Both increases were suppressed by two ferroptotic inhibitors, the lipophilic antioxidant ferrostatin-1 and the membrane-permeable Fe chelator ciclopirox olamine, applied 24 h after the heat shock. These authors also observed a protective effect of D-linoleate (16 h) against the treatment in Synechocystis sp., suggesting that prokaryotic cells exposed to 50°C heat shock could lead to an oxidative Fe-dependent form of cell death with similar characteristics to ferroptosis in the eukaryotic cells (Bogacz and Krauth-Siegel, 2018; Dangol et al., 2018).
Aquatic animals have high levels of PUFA to maintain cell membrane fluidity, especially those living in low-temperature environments (Abele and Puntarulo, 2004); therefore, they are prone to face LP when coping with high Fe concentrations. Numerous studies have analyzed Fe effects on the lipidic fraction of marine organisms, and they could be related to ferroptosis. Recently, Taze et al. (2016) exposed the bivalve Mytilus galloprovincialis to Fe oxide nanoparticles. They observed in the hemocytes a positive correlation between an increase in the DCFH-DA oxidation with LP (measured as thiobarbituric acid reactive substances, TBARS), DNA damage, and protein carbonylation. Also, blue mussel Mytilus sp. gills exposed to both, nano-Fe2O3 particles and a solution of the hydrated FeCl3 for 12 h showed increased LP (measured as malondialdehyde, MDA) as compared to un-exposed animals (Kádár et al., 2010). In another study, the gills and mantle of M. arenaria were analyzed after the exposure of the bivalve to elevated Fe levels (500 µM of Fe as Fe-EDTA) (González et al., 2015). After 9 days of exposure, there was a significant increase in the total Fe and LIP content in the gills. Even more, LIP content represented 40% of the total Fe on day 17. After 17 days of exposure, the DCFH-DA oxidation rate was higher as compared to controls, and TBARS content increased by 1.9- and 3.7-fold over controls on days 9 and 17, respectively. Similar experiments were performed on the digestive glands and significant increases in total Fe, LIP, and TBARS contents were observed after 17 days of Fe incubation (González et al., 2010). These results address the possibility of a ferroptosis disruption in M. arenaria after facing an excess of dissolved Fe. Similar effects were noticed in the mantle. Even more, González and Puntarulo (2016b) showed that the oxidative condition of the digestive gland of the Antarctic limpet Nacella concinna constantly covered by natural Fe-enriched waters (subtidal) was more altered than the intertidal population. Total Fe, LIP content, and lipid damage (assessed as TBARS content) were significantly higher in the subtidal, as compared to the intertidal population (1242 ± 367 and 491 ± 102 pmol/mg FW, respectively). Han et al. (2021) reported an increment in the intracellular ROS levels and a decrease in the neutral lipid content, in the sexual organs of the rotifer Brachionus plicatilis after exposure to 6 and 12 µg/mL of Fe.
The antioxidant defense system
Ferroptosis response can be regulated through the antioxidant defense system, together with metabolism, gene transcription, and protein degradation mechanisms (Chen et al., 2021). Even though ferroptosis can be suppressed by the effect of Fe chelators and lipophilic antioxidants (Stockwell et al., 2017), it can be initiated by a decrease in the antioxidant mechanisms. For example, the early depletion in non-enzymatic antioxidants such as GSH and ascorbate (AH−), was characterized in ferroptotic conditions in plants and animals (Seiler et al., 2008; Skouta et al., 2014; Distéfano et al., 2017). The GSH metabolism is the main pathway limiting ferroptosis. Biochemically, ferroptosis occurs mainly when there is an intracellular GSH depletion and a drop in the glutathione peroxidase 4 (GPx4) activity. Then, lipid peroxides cannot be metabolized by the GPx4-catalyzed reduction reaction, and Fe2+ oxidizes lipids through the Fenton reaction, generating a large amount of ROS (Yang and Stockwell, 2008; Friedmann Angeli et al., 2014). GPx4 is a phospholipid selenium-dependent glutathione peroxidase (GPx) isozyme mainly present in mammalian tissues (Margis et al., 2008). GPx4 is present in the nucleus, cytosol, and mitochondria and bound to membranes in cells (Herbette et al., 2007). This enzyme is also known to be present in fishes and plants; but its connection to ferroptosis has not been established yet (Conrad et al., 2018). Nevertheless, the Fe-dependent cell death process with similar pathways in plants during heat shock and other stressors has already been described. Invertebrates usually express cysteine (Cys)-containing homologs of GPx4 (Ingold and Conrad, 2018). Possible scenarios of ferroptosis in aquatic organisms exposed to Fe could be linked to some of the above-mentioned conditions.
Lipophilic antioxidants enhance the oxidative stability of the cell membranes owing to their ability to protect PUFA from peroxidation and to scavenge free radicals. Among the lipophilic antioxidant compounds, carotenoids, tocopherols, and ubiquinols are the most recognized. Carotenoids represent an important group of pigments in marine environments and terrestrial species (Galasso et al., 2017). They are biosynthesized by autotrophic marine groups: bacteria and archaea, algae, and fungi. Some heterotrophic organisms contain carotenoids, but these are accumulated from food sources or are partly modified through cellular metabolic reactions (Cardoso et al., 2017). Their lipophilic nature allows them to enter the cellular bilayer membrane. Therefore, they can: (i) chelate singlet molecular oxygen (1O2); (ii) change hydroperoxides into more stable compounds; (iii) inhibit free radical oxidation reactions; and (iv) act as Fe and Cu quenchers (Galasso et al., 2017). Since these antioxidants reduce lipidic damage and trap Fe ions, their synthesis in algae and their incorporation in animals result crucial to avoid any scenario of ferroptosis, especially in cold water organisms.
Estévez et al. (2001) evaluated the content of lipophilic antioxidants, both α-tocopherol (α-T) and β-carotene (β-C) in C. vulgaris cells exposed to 5, 90, and 500 µM Fe. Although the content of β-C was not affected, α-T content showed a significant increment with the increase in cellular Fe availability. Robello et al. (2021) also reported a significant increase in the α-T and β-C contents in the lag phase of the development of Fragilaria sp. Cells supplemented with Fe, as compared to the values of controls. Considering lipophilic oxidative ratios, the Fe supplementation produced a significant reduction in the LR•/α-T and LR•/β-C ratios in the lag phase of growth. During the lag phase, the cells seemed well prepared to deal with an excess Fe also in the hydrophobic intracellular environment. Due to an increment in AH− content, the ascorbyl (A•)/AH− ratio, an indicator of the oxidative balance in the hydrophilic medium of the biological systems, was significantly increased in cells in the stationery and lag phases of growth. Antioxidant enzymes also showed significant changes in Fragilaria sp. exposed to Fe. A reduction in the superoxide dismutase (SOD, 36% as compared to controls) activity, catalase (CAT, 72% of control values) content, and nitric oxide generation rate (NO, 60% of control values) was reported in 50 µM Fe supplementation experiments in cells in the lag phase of development.
González et al. (2017) reported a significantly higher activity of the antioxidant enzymes CAT and glutathione transferase (GST) in the Antarctic macroalgae G. skottsbergii collected from Island D with high Fe input as compared to those values in the algae samples collected in Peñón de Pesca (the control area). No significant differences were observed in SOD activity and α-T content in the organisms from both locations. In contrast, the content of AH− and β-C was lower in the macroalgae collected from Island D compared to those measured in Peñón de Pesca. The LR•/α-T, LR•/β-C, and LR•/(α-T + β-C) content ratios (indicators of the oxidative balance in the lipophilic medium of the biological systems) were increased by 2-, 6- and 4-fold, respectively in G. skottsbergii collected from the island, as compared to those algae from the control zone, suggesting that the damaging effect of excess Fe overwhelmed the protection in the macroalgae exposed to high Fe content.
Moreover, Aguilera et al. (2021) observed that the exposure of Synechocystis sp. cells to a temperature of 50°C induced a decrease in GSH and AH− total content. The addition of ferroptosis inhibitors (Fer-1 or CPX) could not prevent the depletion of these antioxidants, suggesting that the reduction of GSH and AH− might be an early outcome in this cell death pathway, as described for Distéfano et al. (2017) in plant ferroptosis. Moreover, cell death was prevented by preincubation with GSH and AH−, suggesting that the decrease in these hydrophilic antioxidants is necessary for the heat shock-induced cell death in Synechocystis sp.
Studies in marine animal tissues, such as the Anguilla gills exposed to silica-coated Fe oxide nanoparticles functionalized with dithiocarbamate (Fe3O4@SiO2/SiDTC, 100 nm; 2.5 mg/L) under in vitro conditions, showed a statistically significant reduction of the total GSH content of the cells after 2 to 72 h of exposure, as compared to control cells. Even more, LP, measured as TBARS, increased significantly after exposure to Fe nanoparticles. Therefore, the antioxidant defense system of the gill was stressed (Srikanth et al., 2014). On the other hand, Li et al. (2009) experimented on exposed embryos and adult medaka Oryzias latipes to nano-Fe particles (average size 30 nm, at doses of 0.5, 5, and 50 µg/mL, actual nano-Fe concentrations of 0.48 ± 0.05, 4.7 ± 0.2, and 46.6 ± 2.7 µg/mL, respectively) and found that the embryos were more sensitive than the adults, regarding the alterations on levels of GSH and MDA. Thus, oxidative damage was only reported in the embryos. Even more, these authors could not find significant changes in the histopathological and morphological alterations or GSH and MDA content in the liver and brain of adult fish. These findings may support the idea that (i) ferroptosis is not developed when GSH levels are not affected by Fe exposure, (ii) immature organisms may be more susceptible to Fe deleterious effects, such as ferroptosis, and (iii) that different tissues may respond distinctly to Fe exposure.
In mammals and invertebrates, two specialized Fe-binding proteins: the extracellular Tf and the intracellular (extracellular in invertebrates) Ft (Winzerling and Law, 1997) provided defense against the toxic effect of Fe and O2 mixtures. The Tf serves to sequester Fe3+ (Kd~10–22 M; Aisen et al., 1978), protecting Fe3+ from hydrolysis at physiological pH, and rendering it unavailable for catalysis of superoxide anion (O2−) formation via Fenton reaction (Gutteridge, 1994). Fts are known as the main Fe storage proteins that can be also used as detoxifying cellular components. The bivalves Hyridella depressa and Margariti fera accumulate Fe in their lysosomes and in calcified concretions (granules) to contribute to the shell formation via Tf and Ft (Simon et al., 2011). Ft has been also shown to function as a participant in shell biomineralization from the bivalve Pinctada fucata (Zhang et al., 2003). Mytilus edulis hemocyte cells with the presence of Ft are considered the major Fe-storage cells in invertebrates (Winston et al., 1996; Ahearn et al., 2004). González and Puntarulo (2011) found that Fe, which is constantly absorbed by the bivalve L. elliptica, is gradually incorporated into Ft. This report also showed that there were non-significant differences in the total amount of Ft in the digestive gland from neither this bivalve nor M. arenaria. In contrast, the Ft isolated from the digestive gland of L. elliptica showed a significantly higher Fe content than the Ft isolated from M. arenaria. These findings suggest that the environmental Fe content is responsible for the Fe amount incorporated into the main cellular storage protein. Ft has also been identified in the oyster Crassostrea gigas (Durand et al., 2002).
To be incorporated into Ft, Fe3+ is reduced to Fe2+, where it is stored as Fe3+ (inert form); nevertheless, by redox reaction with other cellular components (i.e., O2−), Fe3+ from Ft could be reduced to Fe2+, released to the cytoplasm and be incorporated into the LIP. Ft plays a dual role in LIP homeostasis regulating the amount of catalytically active Fe in the cytosol. At high intracellular Fe concentrations, Ft binds Fe to protect cells against its toxicity. In contrast, at low Fe concentrations, Ft releases the metal from its protein core to satisfy the cellular requirements. This release was already proved through in vitro experiments (Winzerling and Law, 1997). However, since Fe is stored as Fe3+, appropriate reductants are required for the mobilization process (Funk et al., 1985). Ft isolated from digestive glands from different mollusk species, a bivalve, and limpets, were exposed to three different AH− (used as a Fe reductant) concentrations (Table 1). In the studied species, Fe was released from the Ft and the Fe liberation rate was significantly increased at higher AH− content duplicating its release in every case and species, which suggests the importance of this mechanism for cellular Fe availability.
Genetic hallmarks of ferroptosis linked to Fe
Ferroptosis is a biological process regulated by multiple genes, including genetic changes in Fe homeostasis and LP. Genes coding for Tf and transferrin receptor (Tfr) control Fe content and modulate ferroptosis due to their capacity to import Fe into cells (Gao et al., 2015); Fe-responsive element binding protein 2 (IREB2) a regulator of Fe metabolism (Dixon et al., 2012); and the machinery for degradation of Ft, known as ferritinophagy (Mancias et al., 2014; Gao et al., 2016; Hou et al., 2016; Wang et al., 2016). Ft is recognized by NCOA4 (Mancias et al., 2014), and this product also modulates ferroptosis sensitivity in some species. Finoshin et al. (2020) studied the Fe metabolic pathways in the processes of sponge plasticity and some known proteins of Fe metabolism, such as the light chain of Ft, IREB2, Zip14 (Fe2+ transporter), Dcytb and STEAP2/3 (membrane metal reductase transporters), ceruloplasmin (Cu-binding glycoprotein with ferroxidase activity), hephaestin (intestinal and ferroxidase), mitochondrial transporter ABCB10, hepcidin/hemochromatosis/hemojuvelin (systemic regulator of Fe metabolism and its regulators), and Tf and Tfr1/2 were not found.
Caspase-3/7 activities were not induced after heat shock (50°C treatment) in Synechocystis sp. In animals, the central executioners of apoptosis are the cysteine-dependent proteases named caspase families implicated in the regulation of several RCD signaling pathways (van Opdenbosch and Lamkanfi, 2019). Other multicellular and unicellular organisms lack true caspases but contain various protein homologs, such as metacaspases and orthocaspases (Klemenčič and Funk, 2018). Most of their functions and regulations have not yet been studied (Klemenčič et al., 2019).
Aguilera et al. (2021) also examined the molecular mechanisms governing ferroptosis in the cyanobacteria Synechocystis sp. cells exposed to 50°C. The studied genes included cyanobacterial GSH synthesis (gshA and gshB) and GSH catabolism (ggt; Narainsamy et al., 2016); and gpx1 and gpx2 are orthologues to human GPx4, essential in animals ferroptosis regulation (Friedmann Angeli et al., 2014). While the three genes gshA, ggt, and gpx1, were upregulated after heat shock exposure, the gpx2 gene was downregulated. The involvement of a pseudo-orthocaspase (SyOC), a prokaryotic caspase-homolog lacking the p10 domain, in the oxidative stress of the cyanobacteria model Synechocystis sp. PCC 6803 was described by Aguilera et al. (2021). To study the in vivo impact of this pseudo-protease during oxidative stress, its gene expression at H2O2 exposure was monitored by real-time-quantitative polymerase chain reaction. Deletion of SyOC led cells with a higher tolerance towards oxidative stress, suggesting that this protein may be involved in a pro-death pathway.
NCOA4 is a nuclear receptor that can be found in different tissues. Arroyo Salazar (2019) studied the expression of NCOA4 in the gonads of the bivalve Crassostrea virginica faced to hydrocarbons exposition. NCOA4 genes displayed a higher significative expression in males than in females (Guévélou et al., 2013). Even more, higher gene expression was found in organisms of both sexes exposed to hydrocarbons, as compared to control values. Even though up to now there are no studies that may link NCOA4 and Ft in mollusks, it is possible to presume a relationship between this gene activity with the oxidative stress generated by exposure to hydrocarbons (Arroyo Salazar, 2019).
Table 2 summarizes the biochemical and genetic hallmarks of ferroptosis in recent literature on mollusks. Much more work is required to understand this complex phenomenon.
Interesting research on the Fe role has been done on aquatic organisms throughout the years; however, no direct relation with ferroptosis was clarified yet. A new approach to this LP-mediated effect is proposed since many of the biomarkers and parameters measured in these organisms (e.g., after exposure to high amounts of Fe, derived from natural or anthropogenic sources), could be directly implicated in triggering ferroptosis. Fe is a metal with a double-edge effect on cells especially depending on its concentration. At low levels, it acts as a micronutrient, often being the central ion in molecules of diverse biological functions, especially in electron transport systems. However, passing a certain threshold level may act as a catalyst for the generation of free radicals. This last Fe function in cells is now of interest to medical surveys, as a new form of cell death, by the triggering of ferroptosis. However, this impact could be also expanded to environmental organisms that face exposure to high Fe concentrations in the medium.
Phytoplankton often dies spontaneously in adverse environmental conditions. Cell death by lysis has been documented in field populations exceeding 50% of phytoplankton growth (Agustí et al., 1998) The induction of autocatalytic programmed cell death (PCD) by biotic or abiotic stresses in prokaryotic and eukaryotic phytoplankton provides one of many reasons behind high lysis rates, independent of viral attack or grazing by heterotrophs (Bidle, 2016). These intrinsic molecular mechanisms could influence the microbial loop changing the flow of photosynthetically fixed organic matter, and associated elements, through the main ecosystem food web pathways, and serves to regulate ocean biogeochemistry (Kirchman, 1999; Bidle and Falkowski, 2004; Bidle, 2015).
On the other hand, RCD has been proposed to optimize differentiation, dynamics, and colony fitness in cyanobacteria (Meeks and Elhai, 2002; Bar-Zeev et al., 2013). Aguilera et al. (2021) found that in response to heat stress (50°C), Synechocystis sp. follows PCD exhibiting biochemical and morphological features that resemble eukaryotic ferroptosis. Moreover, the pathway in Synechocystis sp. PCC 6803 single orthocaspase is characterized by LP and an early decrease of the antioxidants GSH and AH− content (Aguilera et al., 2019). Reduction of GSH content could be a potential hallmark to distinguish ferroptosis-like from other types of PCD (Zhou et al., 2020). As mentioned, this cell death is dependent on Fe availability, and LP, and is inhibited by canonical ferroptosis inhibitors. However, Aguilera et al. (2021) proved that inhibitors and the external addition of antioxidants could not suppress cell death under conditions of high temperature (77°C) or H2O2 (10 mM) exposure. This result suggests that both conditions led to accidental cell death. Still, additional components of the pathway involved in ferroptosis-like processes in cyanobacteria have not yet been identified even though genes related to GSH synthesis and Fe transport are induced after 50°C exposure (Aguilera et al., 2021). The fact of endosymbiotic cyanobacterial origin of chloroplasts and the presence of this Fe-dependent oxidative PCD pathway in cyanobacteria, suggest the evolutionary origin of the role of chloroplast during plant ferroptosis (Distéfano et al., 2017). This observation has not only provided information about the evolution of cell death in unicellular photoautotrophs, but also acknowledges the impact of PCD on the fate of natural phytoplankton assemblages and its role in aquatic biogeochemical cycles (Bidle, 2016).
Studies in biological systems suggest hormesis as an adaptive response of cells and organisms to a moderate stress condition. Hormetic responses involve signaling pathways typically leading to the alteration of the activities of enzymes, such as kinases and deacetylases, and transcription factors that trigger biologically beneficial effects. Fe is not only responsible of ferroptosis conditions, but it is also a hormetic agent in the low-dose range (Galleano et al., 2011; Piloni et al., 2018). Some aquatic organisms exposed to slight sublethal stress conditions trigger a protective response against greater successive challenges. Therefore, the level of stress can lead to a dose-response effect resulting in the so-called hormesis process (Southam and Ehrlich, 1943; Calabrese and Blain, 2011). Thus, hormesis is a biphasic dose-response relationship with contrasting effects of low (with positive/stimulatory responses) vs. high (with negative/inhibitory adverse responses) doses of stress (Agathokleous et al., 2020). The stress conditions known may initiate hormesis comprise exposure to pollutants, metals, agrochemicals, toxins, natural products, ionizing radiation, caloric restriction, hypoxic conditions, ischemic pre-conditioning, and many other provocations (Schmitt et al., 2002; Schulz et al., 2007; Calabrese, 2013; Schmeisser et al., 2013, Chamsi et al., 2019). A temporary up-regulation of endogenous activities of antioxidant enzymes focusing on the improvement in reactive species detoxification has emerged as a feature for many aquatic organisms to tolerate, for example, hypoxia conditions (Oliveira et al., 2018). Nowadays hormesis is fast emerging in plant science research and has large repercussions for risk assessment, stress biology, and agriculture (Agathokleous et al., 2020). In this context, moderate increases in ROS, generated by Fe exposure, during a limited period may lead to gene expression regulation with consequently cytoprotective responses for exposure to subsequent noxious events (Dröge, 2002; Das and Das, 2008). Protective effects of Fe have been reported by Pagano et al. (1996) in sea urchin embryos from the species Paracentrotus lividus and Psammechinus microtuberculatus and embryos of the bivalve M. galloprovincialis exposed to ferric chloride [FeCI3•6H20]. The authors showed that in P. lividus embryos, an addition of Fe3+ low-level concentration (10−8 to 10−7 M) improved larval quality, addressing it to a hormetic effect, as was previously reported for other low-level toxicants (Pagano et al., 1986). Nevertheless, this protective effect was not observed in the M. galloprovincialis embryos.
The diagram shown in Fig. 1 summarizes the reported data about ferroptosis hallmarks (grouped as morphological, biochemical, protein, and genetic characteristics) previously described in vertebrates which have been seen in aquatic organisms. Once Fe is incorporated into the organisms, through ingestion of food or from the dissolved Fe fraction, oxidative stress and damage can be triggered with an increase in ROS generation and a depletion of the antioxidant system leading to ferroptosis. However, if Fe chelators, certain antioxidants, and specifically regulating genes are activated, ferroptosis can be prevented. Other stressors that might affect Fe homeostasis or trigger signaling factors in common with Fe could also be responsible for the induction of ferroptosis or hormesis, depending on the imbalance in the oxidative condition. Thus, the global scenario is even more complicated than it was previously understood regarding the role of Fe as a toxic component in biological systems. Much more research is needed on this subject to improve the life span and survival of aquatic organisms after exposure to natural and anthropogenic adverse conditions.
Figure 1: A summary of ferroptosis hallmarks in aquatic organisms. Writing in blue indicates reported information in aquatic organisms. LP, lipid peroxidation; ROS, reactive oxygen species; Ft, ferritin; GPx4, glutathione peroxidase 4; GSH, reduced glutathione; SyOC, pseudo-orthocaspase (SyOC) gene; gengshA, γ-glutamyl-cysteine ligase gene; ggt, γ-glutamyl transpeptidase gene; gpX1, orthologue to human GPx4 gene; gshB, glutathione synthase gene; gpx2, orthologue to human GPx4 gene.
Funding Statement: This study was supported by grants from the University of Buenos Aires (UBACyT 20020170100199BA), the National Agency of Research, Technological Development and Innovation (ANPCyT) (PICT-2020-SERIEA-03542), and the National Council for Science and Technology (CONICET) (PIP 11220210100183CO). PMG and SP are career investigators (CIC) from CONICET and JC is a fellow from CONICET.
Author Contributions: The authors confirm contribution to the paper as follows: study conception and design: P.M. González and S. Puntarulo; data collection: P.M. González and J. Cabrera; analysis and interpretation of results: P.M. González and J. Cabrera, S. Puntarulo; draft manuscript preparation: P.M. González and J. Cabrera, S. Puntarulo. All authors reviewed the results and approved the final version of the manuscript.
Ethics Approval: Not applicable.
Conflicts of Interest: The authors declare that they have no conflicts of interest to report regarding the present study.
References
Abele D, Puntarulo S (2004). Formation of reactive species and induction of antioxidant defence systems in polar and temperate marine invertebrates and fish. Comparative Biochemistry and Physiology Part A: Molecular & Integrative Physiology 138: 405–415. https://doi.org/10.1016/j.cbpb.2004.05.013 [Google Scholar] [PubMed] [CrossRef]
Adams JB, Palmer F, Staley JT (1992). Rock weathering in deserts: Mobilization and concentration of ferric iron by microorganisms. Geomicrobiology Journal 10: 99–114. https://doi.org/10.1080/01490459209377910 [Google Scholar] [CrossRef]
Agathokleous E, Kitao M, Calabrese EJ (2020). Hormesis: Highly generalizable and beyond laboratory. Trends in Plant Science 25: 1076–1086. https://doi.org/10.1016/j.tplants.2020.05.006 [Google Scholar] [PubMed] [CrossRef]
Aguilera A, Berdum F, Bartoli CG, Steelheart C, Alegre M, Salerno G, Pagnussat GC, Martin MV (2019). Heat stress induces ferroptosis in a photosynthetic prokaryote. bioRxiv. https://doi.org/10.1101/828293 [Google Scholar] [CrossRef]
Aguilera A, Klemenčič M, Sueldo DJ, Rzymski P, Giannuzzi L, Martin MV (2021). Cell death in cyanobacteria: Current understanding and recommendations for a consensus on its nomenclature. Frontiers in Microbiology 12: 631–654. https://doi.org/10.3389/fmicb.2021.631654 [Google Scholar] [PubMed] [CrossRef]
Agustí S, Satta MP, Mura MP, Benavent E (1998). Dissolved esterase activity as a trace of phytoplankton lysis: Evidence of high phytoplankton lysis rates in the Northwestern Mediterranean. Limnology and Oceanography 43: 1836–1849. https://doi.org/10.4319/lo.1998.43.8.1836 [Google Scholar] [CrossRef]
Ahearn GA, Mandal PK, Mandal A (2004). Mechanisms of heavy-metal sequestration and detoxification in crustaceans: A review. Journal of Comparative Physiology 174: 439–452. https://doi.org/10.1007/s00360-004-0438-0 [Google Scholar] [PubMed] [CrossRef]
Aisen P, Leibman A, Zweier J (1978). Stoichiometric and site characteristics of the binding of iron to human transferrin. The Journal of Biological Chemistry 253: 1930–1937. https://doi.org/10.1016/S0021-9258(19)62337-9 [Google Scholar] [CrossRef]
Arroyo Salazar SA (2019). Cambios en los patrones de expresión de genes asociados a la maduración gonadal como respuesta a la exposición por hidrocarburos en Crassostrea virginica. In: Tesis Maestría en Ciencias de la Vida con orientación en Biotecnología Marina, Ensenada, Baja California, México: CICESE. http://cicese.repositorioinstitucional.mx/jspui/handle/1007/3065 [Google Scholar]
Bar-Zeev E, Avishay I, Bidle KD, Berman-Frank I (2013). Programmed cell death in the marine cyanobacterium Trichodesmium mediates carbon and nitrogen export. ISME Journal 7: 2340–2348. https://doi.org/10.1038/ismej.2013.121 [Google Scholar] [PubMed] [CrossRef]
Bidle KD (2015). The molecular ecophysiology of programmed cell death in marine phytoplankton. Annual Review of Marine Science 7: 341–375. https://doi.org/10.1146/annurev-marine-010213-135014 [Google Scholar] [PubMed] [CrossRef]
Bidle KD (2016). Programmed cell death in unicellular phytoplankton. Current Biology 26: R594–R607. https://doi.org/10.1016/j.cub.2016.05.056 [Google Scholar] [PubMed] [CrossRef]
Bidle KD, Falkowski PG (2004). Cell death in planktonic photosynthetic microorganisms. Nature Reviews Microbiology 2: 643–655. https://doi.org/10.1038/nrmicro956 [Google Scholar] [PubMed] [CrossRef]
Bogacz M, Krauth-Siegel RL (2018). Tryparedoxin peroxidase-deficiency commits trypanosomes to ferroptosis-type cell death. eLife 7: e37503. https://doi.org/10.7554/eLife.37503 [Google Scholar] [PubMed] [CrossRef]
Boyd PW, Jickells T, Law CS, Blain S, Boyle EA et al. (2007). Mesoscale iron enrichment experiments 1993–2005: Synthesis and future directions. Science 315: 612–617. https://doi.org/10.1126/science.1131669 [Google Scholar] [PubMed] [CrossRef]
Caccia VG, Millero FJ, Palanques A (2003). The distribution of trace metals in Florida Bay sediments. Marine Pollution Bulletin 46: 1420–1433. https://doi.org/10.1016/S0025-326X(03)00288-1 [Google Scholar] [PubMed] [CrossRef]
Calabrese EJ (2013). Hormetic mechanisms. Critical Reviews in Toxicology 43: 580–606. https://doi.org/10.3109/10408444.2013.808172 [Google Scholar] [PubMed] [CrossRef]
Calabrese EJ, Blain RB (2011). The hormesis database: The occurrence of hormetic dose responses in the toxicological literature. Regulatory Toxicology and Pharmacology 61: 73–81. https://doi.org/10.1016/j.yrtph.2011.06.003 [Google Scholar] [PubMed] [CrossRef]
Cardoso LA, Karp SG, Vendruscolo F, Kanno KY, Zoz LI, Carvalho JC (2017). Biotechnological production of carotenoids and their applications in food and pharmaceutical products. Carotenoids 8, 125141. [Google Scholar]
Chamsi O, Pinelli E, Faucon B, Perrault A, Lacroix L, Sánchez Pérez JM, Charcosset JY (2019). Effects of herbicide mixtures on freshwater microalgae with the potential effect of a safener. Annual Limnology International Journal of Limnology 55: 3. https://doi.org/10.1051/limn/2019002 [Google Scholar] [CrossRef]
Chavez-Crooker P, Garrido N, Ahearn GA (2001). Copper transport by lobster hepatopancreatic epithelial cells separated by centrifugal elutriation: Measurements with the fluorescent dye. Phen Green. The Journal of Experimental Biology 204: 1433–1444. https://doi.org/10.1242/jeb.204.8.1433 [Google Scholar] [PubMed] [CrossRef]
Chen X, Comish PB, Tang D, Kang R (2021). Characteristics and biomarkers of ferroptosis. Frontiers in Cell and Developmental Biology 9: 637162. https://doi.org/10.3389/fcell.2021.637162 [Google Scholar] [PubMed] [CrossRef]
Chen X, Yu C, Kang R, Tang D (2020). Iron metabolism in ferroptosis. Frontiers in Cell and Developmental Biology 8: 590226. https://doi.org/10.3389/fcell.2020.590226 [Google Scholar] [PubMed] [CrossRef]
Conrad M, Kagan VE, Bayir H, Pagnussat GC, Head B, Traber MG, Stockwell BR (2018). Regulation of lipid peroxidation and ferroptosis in diverse species. Genes and Development 32: 602–619. https://doi.org/10.1101/gad.314674.118 [Google Scholar] [PubMed] [CrossRef]
Dangol S, Chen Y, Hwang BK, Jwa NS (2018). Iron- and reactive oxygen species dependent ferroptotic cell death in rice—Magnaporthe oryzae interactions. Plan Cell 31: 189–209. https://doi.org/10.1105/tpc.18.00535 [Google Scholar] [PubMed] [CrossRef]
Das M, Das DK (2008). Molecular mechanism of preconditioning. IUBMB Life 60: 199–203. https://doi.org/10.1002/(ISSN)1521-6551 [Google Scholar] [CrossRef]
Distéfano AM, Martin MV, Córdoba JP, Bellido AM, D’Ippólito S et al. (2017). Heat stress induces ferroptosis-like cell death in plants. Journal of Cell Biology 216: 463–476. https://doi.org/10.1083/jcb.201605110 [Google Scholar] [PubMed] [CrossRef]
Dixon SJ, Lemberg KM, Lamprecht MR, Skouta R, Zaitsev EM et al. (2012). Ferroptosis: An iron-dependent form of nonapoptotic cell death. Cell 149: 1060–1072. https://doi.org/10.1016/j.cell.2012.03.042 [Google Scholar] [PubMed] [CrossRef]
Dixon SJ, Stockwell BR (2014). The role of iron and reactive oxygen species in cell death. Nature Chemical Biology 10: 9–17. https://doi.org/10.1038/nchembio.1416 [Google Scholar] [PubMed] [CrossRef]
Dröge W (2002). Free radicals in the physiological control of cell function. Physiological Reviews 82: 47–95. https://doi.org/10.1152/physrev.00018.2001 [Google Scholar] [PubMed] [CrossRef]
Durand JP, Goudard F, Barbot C, Pieri J, Fowler SW, Cotret O (2002). Ferritin and hemocyanin: 210Po molecular traps in marine fish, oyster and lobster. Marine Ecology Progress Series 233: 199–205. https://doi.org/10.3354/meps233199 [Google Scholar] [CrossRef]
Estévez MS, Malanga G, Puntarulo S (2001). Iron-dependent oxidative stress in Chlorell vulgaris. Plant Science 161: 9–17. https://doi.org/10.1016/S0168-9452(01)00364-8 [Google Scholar] [CrossRef]
Estévez S, Puntarulo S (2005). Nitric oxide generation upon growth of Antarctic Chlorella sp. cells. Physiologia Plantarum 125: 192–201. https://doi.org/10.1111/j.1399-3054.2005.00561.x [Google Scholar] [CrossRef]
Fan J, Cui Y, Wan M, Wang W, Li Y (2014). Lipid accumulation and biosynthesis genes response of the oleaginous Chlorella pyrenoidosa under three nutrition stressors. Biotechnology for Biofuels 7: 17. https://doi.org/10.1186/1754-6834-7-17 [Google Scholar] [PubMed] [CrossRef]
Feng H, Schorpp K, Jin J, Yozwiak CE, Hoffstrom BG et al. (2020). Transferrin receptor is a specific ferroptosis marker. Cell Reports 30: 3411–3423.e7. https://doi.org/10.1016/j.celrep.2020.02.049 [Google Scholar] [PubMed] [CrossRef]
Finoshin AD, Adameyko KI, Mikhailov KV, Kravchuk OI, Georgiev AA et al. (2020). Iron metabolic pathways in the processes of sponge plasticity. PLoS One 15: e0228722. https://doi.org/10.1371/journal.pone.0228722 [Google Scholar] [PubMed] [CrossRef]
Friedmann Angeli JP, Schneider M, Proneth B, Tyurina YY, Tyurin VA et al. (2014). Inactivation of the ferroptosis regulator Gpx4 triggers acute renal failure in mice. Nature Cell Biology 16: 1180–1191. https://doi.org/10.1038/ncb3064 [Google Scholar] [PubMed] [CrossRef]
Funk F, Lenders JP, Crichton RR, Schneider W (1985). Reductive mobilization of ferritin iron. European Journal of Biochemistry 152: 167–172. https://doi.org/10.1111/j.1432-1033.1985.tb09177.x [Google Scholar] [PubMed] [CrossRef]
Galasso C, Corinaldesi C, Sansone C (2017). Carotenoids from marine organisms: Biological functions and industrial applications. Antioxidants 6: 96. https://doi.org/10.3390/antiox6040096 [Google Scholar] [PubMed] [CrossRef]
Galleano M, Tapia G, Puntarulo S, Varela P, Videla LA, Fernandez V (2011). Liver preconditioning induced by iron in a rat model of ischemia/reperfusion. Life Sciences 89: 221–228. https://doi.org/10.1016/j.lfs.2011.06.005 [Google Scholar] [PubMed] [CrossRef]
Gao M, Monian P, Pan Q, Zhang W, Xiang J, Jiang X (2016). Ferroptosis is an autophagic cell death process. Cell Research 26: 1021–1032. https://doi.org/10.1038/cr.2016.95 [Google Scholar] [PubMed] [CrossRef]
Gao M, Monian P, Quadri N, Ramasamy R, Jiang X (2015). Glutaminolysis and transferrin regulate ferroptosis. Molecular Cell 59: 298–308. https://doi.org/10.1016/j.molcel.2015.06.011 [Google Scholar] [PubMed] [CrossRef]
Ghafari M, Rashidi B, Haznedaroglu BZ (2018). Effects of macro and micronutrients on neutral lipid accumulation in oleaginous microalgae. Biofuels 9: 147–156. https://doi.org/10.1080/17597269.2016.1221644 [Google Scholar] [CrossRef]
González PM, Abele D, Puntarulo S (2010). Exposure to excess of iron in vivo affects oxidative status in the bivalve Mya arenaria. Comparative Biochemistry and Physiology C 152: 167–174. https://doi.org/10.1016/j.cbpc.2010.04.006 [Google Scholar] [PubMed] [CrossRef]
González PM, Abele D, Puntarulo S (2012b). A kinetic approach to assess oxidative metabolism related features in the bivalve Mya arenaria. Theory in Biosciences 131: 253–264. https://doi.org/10.1007/s12064-012-0159-y [Google Scholar] [PubMed] [CrossRef]
González PM, Abele D, Puntarulo S (2015). Oxidative status of respiratory tissues of the bivalve Mya arenaria after exposure to excess dissolved iron. Marine and Freshwater Behaviour and Physiology 48: 103–116. https://doi.org/10.1080/10236244.2015.1004839 [Google Scholar] [CrossRef]
González PM, Puntarulo S (2016a). Seasonality and toxins effects on oxidative/nitrosative metabolism in digestive glands of the bivalve Mytilus edulis platensis. Comparative Biochemistry and Physiology Part A: Molecular & Integrative Physiology 200: 79–86. https://doi.org/10.1016/j.cbpa.2016.04.011 [Google Scholar] [PubMed] [CrossRef]
González PM, Deregibus D, Malanga G, Campana GL, Zacher K, Quartino ML, Puntarulo S (2017). Oxidative balance in macroalgae from Antarctic waters. Possible role of Fe. Journal of Experimental Marine Biology and Ecology 486: 379–386. https://doi.org/10.1016/j.jembe.2016.10.018 [Google Scholar] [CrossRef]
González PM, Piloni NE, Puntarulo S (2012c). Iron overload and lipid peroxidation in biological systems. In: Catalá A, (ed.Lipid Peroxidation, vol. 4, pp. 89–108. Rijeka, Croatia: InTech. [Google Scholar]
González PM, Puntarulo S (2011). Iron and nitrosative metabolism in the Antarctic mollusc Laternula elliptica. Comparative Biochemistry and Physiology C 153: 243–250. https://doi.org/10.1016/j.cbpc.2010.11.003 [Google Scholar] [PubMed] [CrossRef]
González PM, Puntarulo S (2016b). Fe, oxidative and nitrosative metabolism in the Antarctic limpet Nacella concinna. Comparative Biochemistry and Physiology A 200: 56–63. https://doi.org/10.1016/j.cbpa.2016.04.007 [Google Scholar] [PubMed] [CrossRef]
González PM, Wilhelms-Dick D, Abele D, Puntarulo S (2012a). Iron in coastal marine ecosystems. Role in oxidative stress. In: Abele D, Zenteno-Savin T, Vazquez-Medina J, (eds.Oxidative Stress in Aquatic Ecosystems, vol. 8, pp. 115–126. West Sussex, UK: Blackwell Publishing Ltd. [Google Scholar]
Gutteridge JMC (1994). Biological origin of free radicals, and mechanisms of antioxidant protection. Chemical-Biological Interactions 91: 133–140. https://doi.org/10.1016/0009-2797(94)90033-7 [Google Scholar] [PubMed] [CrossRef]
Guévélou E, Huvet A, Galindo-Sánchez CE, Milan M, Quillien V, Daniel JY, Corporeau C (2013). Sex-specific regulation of AMP-activated protein kinase (AMPK) in the pacific oyster crassostrea gigas. Biology of Reproduction 89: 1–15. https://doi.org/10.1095/biolreprod.113.109728 [Google Scholar] [PubMed] [CrossRef]
Han C, Kim HJ, Lee JS, Sakakura Y, Hagiwara A (2021). Species-specific effects of iron on temperate and tropical marine rotifers in reproduction, lipid and ROS metabolisms. Chemosphere 277: 130317. https://doi.org/10.1016/j.chemosphere.2021.130317 [Google Scholar] [PubMed] [CrossRef]
Herbette S, Roeckel-Drevet P, Drevet JR (2007). Seleno-independent glutathione peroxidases. FEBS Journal 274: 2163–2180. https://doi.org/10.1111/j.1742-4658.2007.05774.x [Google Scholar] [PubMed] [CrossRef]
Hider RC, Kong XL (2011). Glutathione: A key component of the cytoplasmic labile iron pool. Bio Metals 24: 1179–1187. https://doi.org/10.1007/s10534-011-9476-8 [Google Scholar] [PubMed] [CrossRef]
Hider RC, Kong X (2013). Iron speciation in the cytosol: An overview. Dalton Transaction 42: 3220–3229. https://doi.org/10.1039/C2DT32149A [Google Scholar] [PubMed] [CrossRef]
Hou W, Xie Y, Song X, Sun X, Lotze MT, Zeh HJIII, Kang R, Tang D (2016). Autophagy promotes ferroptosis by degradation of ferritin. Autophagy 12: 1425–1428. https://doi.org/10.1080/15548627.2016.1187366 [Google Scholar] [PubMed] [CrossRef]
Hummel SG, Fischer AJ, Martin SM, Schafer FQ, Buettner GR (2006). Nitric oxide as a cellular antioxidant: A little goes a long way. Free Radical Biology and Medicine 40: 501–506. https://doi.org/10.1016/j.freeradbiomed.2005.08.047 [Google Scholar] [PubMed] [CrossRef]
Hutchins D, Witter A, Butler A, Luther G (1999). Competition among marine phytoplankton for different chelated iron species. Nature 400: 858–861. https://doi.org/10.1038/23680 [Google Scholar] [CrossRef]
Ingold I, Conrad M (2018). Oxidative stress, selenium redox systems including GPX/TXNRD families. In: Selenium, pp. 111–135. Springer. https://doi.org/10.1007/978-3-319-95390-8_6 [Google Scholar] [CrossRef]
Juneja A, Ceballos RM, Murthy GS (2013). Effects of environmental factors and nutrient availability on the biochemical composition of algae for biofuels production: A review. Energies 6: 4607–4638. https://doi.org/10.3390/en6094607 [Google Scholar] [CrossRef]
Kirchman DL (1999). Phytoplankton death in the sea. Nature 398: 293–294. https://doi.org/10.1038/18570 [Google Scholar] [CrossRef]
Klemenčič M, Asplund-Samuelsson J, Dolinar M, Funk C (2019). Phylogenetic distribution and diversity of bacterial pseudo-orthocaspases underline their putative role in photosynthesis. Frontiers in Plant Science 10: 293. https://doi.org/10.3389/fpls.2019.00293 [Google Scholar] [PubMed] [CrossRef]
Klemenčič M, Funk C (2018). Structural and functional diversity of caspase homologues in non-metazoan organisms. Protoplasma 255: 387–397. https://doi.org/10.1007/s00709-017-1145-5 [Google Scholar] [PubMed] [CrossRef]
Koppenol WH, Bounds PL (2011). Redox-active metals: Iron and copper. In: Pantopoulos K, Schipper HM, (eds.Principles of Free Radical Biomedicine, vol. 1, pp. 91–111. New York: Nova Science Publishers, Inc, Hauppage. [Google Scholar]
Koppenol WH, Hider RH (2019). Iron and redox cycling. Do’s and don’ts. Free Radical Biology and Medicine 133: 3–10. https://doi.org/10.1016/j.freeradbiomed.2018.09.022 [Google Scholar] [PubMed] [CrossRef]
Krachler R, Krachler R, Valda A, Keppler BK (2019). Natural iron fertilization of the coastal ocean by blackwater rivers. Science of the Total Environment 656: 952–958. https://doi.org/10.1016/j.scitotenv.2018.11.423 [Google Scholar] [PubMed] [CrossRef]
Kuang F, Liu J, Tang D, Kang R (2020). Oxidative damage and antioxidant defense in ferroptosis. Frontiers in Cell and Developmental Biology 8: 586578. https://doi.org/10.3389/fcell.2020.586578 [Google Scholar] [PubMed] [CrossRef]
Kurz T, Terman A, Brunk UT (2007). Autophagy, ageing and apoptosis: The role of oxidative stress and lysosomal iron. Archives in Biochemistry and Biophysics 462: 220–230. https://doi.org/10.1016/j.abb.2007.01.013 [Google Scholar] [PubMed] [CrossRef]
Kádár E, Lowe DM, Solé M, Fisher AS, Jha AN, Readman JW, Hutchinson TH (2010). Uptake and biological responses to nano-Fe versus soluble FeCl3 in excised mussel gills. Analytical and Bioanalytical Chemistry 396: 657–666. https://doi.org/10.1007/s00216-009-3191-0 [Google Scholar] [PubMed] [CrossRef]
Li C, Zhang Y, Liu J, Kang R, Klionsky DJ, Tang D (2021). Mitochondrial DNA stress triggers autophagy-dependent ferroptotic death. Autophagy 17: 948–960. https://doi.org/10.1080/15548627.2020.1739447 [Google Scholar] [PubMed] [CrossRef]
Li H, Zhou Q, Wu Y, Fu J, Wang T, Jiang G (2009). Effects of water borne nano-iron on medaka (Oryzias latipesAntioxidant enzymatic activity, lipid peroxidation and histopathology. Ecotoxicology and Environmental Safety 72: 684–692. https://doi.org/10.1016/j.ecoenv.2008.09.027 [Google Scholar] [PubMed] [CrossRef]
Liu ZY, Wang GC, Zhou BC (2008). Effect of iron on growth and lipid accumulation in Chlorella vulgaris. Bioresource Technology 99: 4717–4722. https://doi.org/10.1016/j.biortech.2007.09.073 [Google Scholar] [PubMed] [CrossRef]
Lu J, Xu F, Lu H (2020). LncRNA PVT1 regulates ferroptosis through miR-214-mediated TFR1 and p53. Life Sciences 260: 118305. https://doi.org/10.1016/j.lfs.2020.118305 [Google Scholar] [PubMed] [CrossRef]
Luo CS, Liang JR, Lin Q, Li C, Bowler C, Anderson DM, Wang P, Wang XW, Gao YH (2014). Cellular responses associated with ROS production and cell fate decision in early stress response to iron limitation in the diatom Thalassiosira pseudonana. Journal of Proteome Research 13: 5510–5523. https://doi.org/10.1021/pr5004664 [Google Scholar] [PubMed] [CrossRef]
Lushchak VI (2011). Environmentally induced oxidative stress in aquatic animals. Aquatic Toxicology 101: 13–30. https://doi.org/10.1016/j.aquatox.2010.10.006 [Google Scholar] [PubMed] [CrossRef]
Mancias JD, Wang X, Gygi SP, Harper JW, Kimmelman AC (2014). Quantitative proteomics identifies NCOA4 as the cargo receptor mediating ferritinophagy. Nature 509: 105–109. https://doi.org/10.1038/nature13148 [Google Scholar] [PubMed] [CrossRef]
Margis R, Dunand C, Teixeira FK, Margis-Pinheiro M (2008). Glutathione peroxidase family—an evolutionary overview. FEBS Journal 275: 3959–3970. https://doi.org/10.1111/j.1742-4658.2008.06542.x [Google Scholar] [PubMed] [CrossRef]
Martin JH, Fitzwater SE (1988). Iron deficiency limits phytoplankton growth in the north-east Pacific subarctic. Nature 331: 341–343. https://doi.org/10.1038/331341a0 [Google Scholar] [CrossRef]
Meeks JC, Elhai J (2002). Regulation of cellular differentiation in filamentous cyanobacteria in free-living and plant-associated symbiotic growth states. Microbiology and Molecular Biology Reviews 66: 94–121. https://doi.org/10.1128/MMBR.66.1.94-121.2002 [Google Scholar] [PubMed] [CrossRef]
Moore RM, Milley JE, Chatt A (1984). The potential for biological mobilization of trace elements from aeolian dust and its importance in the case of iron. Oceanologica Acta 7: 221–228. https://archimer.ifremer.fr/doc/00113/22423/20119.pdf [Google Scholar]
Narainsamy K, Farci S, Braun E, Junot C, Cassier-Chauvat C, Chauvat F (2016). Oxidative-stress detoxification and signalling in cyanobacteria: The crucial glutathione synthesis pathway supports the production of ergothioneine and ophthalmate. Molecular Biology 100: 15–24. https://doi.org/10.1111/mmi.13296 [Google Scholar] [PubMed] [CrossRef]
Nodwell LM, Price NM (2001). Direct use of inorganic colloidal iron by marine mixotrophic phytoplankton. Limnology and Oceanography 46: 765–777. https://doi.org/10.4319/lo.2001.46.4.0765 [Google Scholar] [CrossRef]
Nuester J, Shema S, Vermont A, Fields DM, Twining BS (2014). The regeneration of highly bioavailable iron by meso- and microzooplankton. Limnology and Oceanography 59: 1399–1409. https://doi.org/10.4319/lo.2014.59.4.1399 [Google Scholar] [CrossRef]
Oliveira MF, Geihs MA, França TFA, Moreira DC, Hermes-Lima M (2018). Is “Preparation for Oxidative Stress” a case of physiological conditioning hormesis? Frontiers in Physiology 9: 945. https://doi.org/10.3389/fphys.2018.00945 [Google Scholar] [PubMed] [CrossRef]
Pagano G, Cipollaro M, Corsale G, Esposito A, Ragucci E, Giordano GG, Trieff NM (1986). The sea urchin: Bioassay for the assessment of damage from environmental contaminants. In: Cairns Jr J (ed.Community Toxicity Testing, vol. 5, pp. 66–92. Philadelphia: Association for Standard Testing and Materials. [Google Scholar]
Pagano G, His E, Beiras R, de Biase A, Korkina LG et al. (1996). Cytogenetic, developmental, and biochemical effects of aluminum, iron, and their mixture in sea urchins and mussels. Archives of Environmental Contamination and Toxicology 31: 466–474. https://doi.org/10.1007/BF00212429 [Google Scholar] [PubMed] [CrossRef]
Piloni NE, Caro AA, Puntarulo S (2018). Iron overload prevents oxidative damage to rat brain after chlorpromazine administration. BioMetals 31: 561–570. https://doi.org/10.1007/s10534-018-0104-8 [Google Scholar] [PubMed] [CrossRef]
Rana MS, Bhushan S, Sudhakar DR, Prajapati SK (2020). Effect of iron oxide nanoparticles on growth and biofuel potential of Chlorella spp. Algal Research 49: 101942. https://doi.org/10.1016/j.algal.2020.101942 [Google Scholar] [CrossRef]
Regoli F, Frenzilli G, Bocchetti R, Annarumma F, Scarcelli V, Fattorini D, Nigro M (2004). Time-course variations of oxyradical metabolism, DNA integrity and lysosomal stability in mussels, Mytilus galloprovincialis, during a field translocation experiment. Aquatic Toxicology 68: 167–178. https://doi.org/10.1016/j.aquatox.2004.03.011 [Google Scholar] [PubMed] [CrossRef]
Riegman M, Sagie L, Galed C, Levin T, Steinberg N et al. (2020). Ferroptosis occurs through an osmotic mechanism and propagates independently of cell rupture. Nature Cell Biology 22: 1042–1048. https://doi.org/10.1038/s41556-020-0565-1 [Google Scholar] [PubMed] [CrossRef]
Robello E, Galatro A, Puntarulo S (2021). Oxidative/nitrosative stress evaluation Fragilaria sp. cultures (Antarctic Diatom) exposed to moderated iron supplementation. Current Topics in Phytochemistry 17: 93–106. http://researchtrends.net/tia/abstract.asp?in=0&vn=17&tid=24&aid=6875&pub=2021 [Google Scholar]
Sato M, Takeda S, Furuya K (2007). Iron regeneration and organic iron (III)-binding ligand production during in situ zooplankton grazing experiment. Marine Chemistry 106: 471–488. https://doi.org/10.1016/j.marchem.2007.05.001 [Google Scholar] [CrossRef]
Schmeisser S, Schmeisser K, Weimer S, Groth M, Priebe S et al. (2013). Mitochondrial hormesis links low-dose arsenite exposure to lifespan extension. Aging Cell 12: 508–517. https://doi.org/10.1111/acel.12076 [Google Scholar] [PubMed] [CrossRef]
Schmitt E, Lehmann L, Metzler M, Stopper H (2002). Hormonal and genotoxic activity of resveratrol. Toxicology Letters 136: 133–142. https://doi.org/10.1016/S0378-4274(02)00290-4 [Google Scholar] [PubMed] [CrossRef]
Schulz TJ, Zarse K, Voigt A, Urban N, Birringer M, Ristow M (2007). Glucose restriction extends Caenorhabditis elegans life span by inducing mitochondrial respiration and increasing oxidative stress. Cell Metabolism 6: 280–293. https://doi.org/10.1016/j.cmet.2007.08.011 [Google Scholar] [PubMed] [CrossRef]
Seiler A, Schneider M, Förster H, Roth S, Wirth EK et al. (2008). Glutathione peroxidase 4 senses and translates oxidative stress into 12/15-lipoxygenase dependent- and AIF-mediated cell death. Cell Metabolism 8: 237–248. https://doi.org/10.1016/j.cmet.2008.07.005 [Google Scholar] [PubMed] [CrossRef]
Shaked Y, Kustka AB, Morel FMM (2005). A general kinetic model for iron acquisition by eukaryotic phytoplankton. Limnology and Oceanography 50: 872–882. https://doi.org/10.4319/lo.2005.50.3.0872 [Google Scholar] [CrossRef]
Simon O, Floriani M, Cavalie I, Camilleri V, Adam C, Gilbin R, Garnier-Laplace J (2011). Internal distribution of uranium and associated genotoxic damages in the chronically exposed bivalve Corbicula fluminea. Journal of Environmental Radioactivity 102: 766–773. https://doi.org/10.1016/j.jenvrad.2011.04.004 [Google Scholar] [PubMed] [CrossRef]
Skouta R, Dixon SJ, Wang J, Dunn DE, Orman M et al. (2014). Ferrostatins inhibit oxidative lipid damage and cell death in diverse disease models. Journal of American Chemical Society 136: 4551–4556. https://doi.org/10.1021/ja411006a [Google Scholar] [PubMed] [CrossRef]
Southam CM, Ehrlich J (1943). Effects of extract of western red-cedar heartwood on certain wood-decaying fungi in culture. Phytopathology 33: 517–524. https://doi.org/10.1139/W08-161 [Google Scholar] [PubMed] [CrossRef]
Srikanth K, Ahmad I, Rao JV, Trindade T, Duarte AC, Pereira E (2014). Modulation of glutathione and its dependent enzymes in gill cells of Anguilla anguilla exposed to silica coated iron oxide nanoparticles with or without mercury co-exposure under in vitro condition. Comparative Biochemistry and Physiology Part C: Toxicology & Pharmacology 162: 7–14. https://doi.org/10.1016/j.cbpc.2014.02.007 [Google Scholar] [PubMed] [CrossRef]
Stockwell BR, Friedmann Angeli JP, Bayir H, Bush AI, Conrad M et al. (2017). Ferroptosis: A regulated cell death nexus linking metabolism, redox biology, and disease. Cell 171: 273–285. https://doi.org/10.1016/j.cell.2017.09.021 [Google Scholar] [PubMed] [CrossRef]
Stohs SJ, Bagchi D (1995). Oxidative mechanisms in the toxicity of metal ions. Free Radical Biology and Medicine 8: 321–336. https://doi.org/10.1016/0891-5849(94)00159-H [Google Scholar] [PubMed] [CrossRef]
Taze C, Panetas I, Kalogiannis S, Feidantsis K, Gallios GP, Kastrinaki G, Konstandopoulos AG, Václavíková M, Ivanicova L, Kaloyianni M (2016). Toxicity assessment and comparison between two types of iron oxide nanoparticles in Mytilus galloprovincialis. Aquatic Toxicology 172: 9–20. https://doi.org/10.1016/j.aquatox.2015.12.013 [Google Scholar] [PubMed] [CrossRef]
Thamatrakoln K, Korenovska O, Kalani Niheu A, Bidle KD (2012). Whole-genome expression analysis reveals a role for death-related genes in stress acclimation of the diatom Thalassiosira pseudonana. Environmental Microbiology 14: 67–81. https://doi.org/10.1111/j.1462-2920.2011.02468.x [Google Scholar] [PubMed] [CrossRef]
van Opdenbosch N, Lamkanfi M (2019). Caspases in cell death, inflammation, and disease. Immunity 50: 1352–1364. https://doi.org/10.1016/j.immuni.2019.05.020 [Google Scholar] [PubMed] [CrossRef]
Wang YQ, Chang SY, Wu Q, Gou YJ, Jia L et al. (2016). The protective role of mitochondrial ferritin on erastin-induced ferroptosis. Frontier in Aging Neuroscience 8: 308. https://doi.org/10.3389/fnagi.2016.00308 [Google Scholar] [PubMed] [CrossRef]
Wang Y, Liu Y, Liu J, Kang R, Tang D (2020). NEDD4L-mediated LTF protein degradation limits ferroptosis. Biochemical and Biophysical Research Communications 531: 581–587. https://doi.org/10.1016/j.bbrc.2020.07.032 [Google Scholar] [PubMed] [CrossRef]
Watson AJ (2001). Iron limitation in the oceans. In: Turner DR, Hunter KA, (eds.The Biogeochemistry of Iron in Seawater. vol. 1, pp. 85–121. New York: John Wiley and Sons. [Google Scholar]
Winston GW, Moore MN, Kirchín MA, Soverchia C (1996). Production of reactive oxygen species by hemocytes from the marine mussel, Mytilus edulis: Lysosomal localization and effect of xenobiotics. Comparative Biochemistry and Physiology Part C: Pharmacology, Toxicology and Endocrinology 113: 221–229. https://doi.org/10.1016/0742-8413(95)02091-8 [Google Scholar] [PubMed] [CrossRef]
Winzerling JJ, Law JH (1997). Comparative nutrition of iron and copper. Annual Review of Nutrition 17: 501–526. https://doi.org/10.1146/annurev.nutr.17.1.501 [Google Scholar] [PubMed] [CrossRef]
Yang WS, Stockwell BR (2008). Synthetic lethal screening identifies compounds activating iron-dependent, nonapoptotic cell death in oncogenic-RAS harboring cancer cells. Chemistry and Biology 15: 234–245. https://doi.org/10.1016/j.chembiol.2008.02.010 [Google Scholar] [PubMed] [CrossRef]
1000. Yang WS, Stockwell BR (2016). Ferroptosis: Death by lipid peroxidation. Trends in Cell Biology 26: 165–176. https://doi.org/10.1016/j.tcb.2015.10.014 [Google Scholar] [PubMed] [CrossRef]
Zhang Y, Meng Q, Jiang T, Wang H, Xie L, Zhang R (2003). A novel ferritin subunit involved in shell formation from the pearl oyster (Pinctada fucata). Comparative Biochemistry and Physiology Part B: Biochemistry and Molecular Biology 135: 43–54. https://doi.org/10.1016/S1096-4959(03)00050-2 [Google Scholar] [PubMed] [CrossRef]
Zhou T, Cao H, Zheng J, Teng F, Wang X, Lou K, Zhang X, Tao Y (2020). Suppression of water-bloom cyanobacterium Microcystis aeruginosa by algaecide hydrogen peroxide maximized through programmed cell death. Journal of Hazardous Materials 393: 122394. https://doi.org/10.1016/j.jhazmat.2020.122394 [Google Scholar] [PubMed] [CrossRef]
Cite This Article
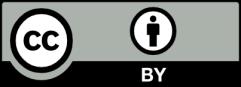
This work is licensed under a Creative Commons Attribution 4.0 International License , which permits unrestricted use, distribution, and reproduction in any medium, provided the original work is properly cited.