Open Access
REVIEW
Intracellular life of protozoan Toxoplasma gondii: Parasitophorous vacuole establishment and survival strategies
1 Laboratório de Ultraestrutura Celular Hertha Meyer, Instituto de Biofísica Carlos Chagas Filho, Universidade Federal do Rio de Janeiro, Rio de Janeiro, Brazil
2 Núcleo Multidisciplinar de Pesquisa UFRJ–Xerém em Biologia, Campus Duque de Caxias, Universidade Federal do Rio de Janeiro, Duque de Caxias, Brazil
3 Laboratório de Quimioterapia de Protozoários Egler Chiari, Departamento de Parasitologia, Universidade Federal de Minas Gerais, Belo Horizonte, Brazil
* Corresponding Author: ERICA S. MARTINS-DUARTE. Email:
BIOCELL 2023, 47(4), 929-950. https://doi.org/10.32604/biocell.2023.026629
Received 16 September 2022; Accepted 12 December 2022; Issue published 08 March 2023
Abstract
Toxoplasma gondii is a protozoan of worldwide distribution and the agent of toxoplasmosis. It is estimated that 30%–50% of the world population could be infected with this parasite. Although the infection in immunocompetent individuals is mostly asymptomatic, the disease in immunosuppressed and pregnant is a risk condition. As a member of the phylum Apicomplexa, T. gondii has an obligatory intracellular lifestyle; therefore, invading a host cell and establishing it inside a parasitophorous vacuole (PV) are mandatories for the survival of this parasite. The construction of a perfect intracellular niche for T. gondii requires the secretion of an arsenal of proteins from unique secretory organelles. These proteins will remodel the vacuolar environment and the host cell organization and functions, allowing the parasite to access essential nutrients and stay “invisible” inside a host cell. In the present review, we will discuss the main steps involved in the PV formation and its differentiation to tissue cyst, focusing mainly on the strategies employed in the acquisition of nutrients and proteins involved in host cell modification.Keywords
Toxoplasma gondii is the causative agent of toxoplasmosis, a disease with worldwide distribution affecting about a third of the world population. Some regions of Central and South America have even reached up to 90% infection rate (Pappas et al., 2009). T. gondii infection occurs through the ingestion of food or water contaminated with oocysts (containing sporozoites), which are excreted in the feces of infected felids (the definitive hosts of T. gondii), or by the ingestion of tissue cysts with bradyzoites in raw or undercooked meat from chronically infected animals. After ingestion of infectious forms, sporozoites and bradyzoites are released in the gastrointestinal tract and infect the cells of the mucosa of the small intestine, where they differentiate into a fast-replicating form called tachyzoite. The latter is responsible for the acute phase of the disease and for the dissemination of the infection from the initial site of entrance in the small intestine to other tissues (Halonen and Weiss, 2013; Attias et al., 2020).
Under the pressure of the immune system, tachyzoite differentiate into bradyzoite, the slow-growing form, which resides inside an intracellular tissue cyst and persists latently through the whole life of infected individuals. Although T. gondii can infect any nucleated cell, it shows a tropism for muscle and central nervous system (CNS) tissues that usually have a high burden of cysts (Blader et al., 2015). All drugs used for the treatment of toxoplasmosis are only active against the acute stage of disease and there is no treatment available for chronic infection (Dunay et al., 2018).
In immunocompetent individuals, the infection is mostly asymptomatic and converts unnoticed into the chronic form of the disease (Montoya and Liesenfeld, 2004). The symptoms in immunocompetent individuals are generally mild and nonspecific, but some individuals may have the ocular form of the disease. In immunosuppressed individuals, however, T. gondii infection is a risk condition, with the site of parasitic tropism being the CNS. The primo-infection, or reactivation of the disease, usually causes encephalitis in these individuals, and if not diagnosed and treated in time, can cause the patient’s death or permanent sequelae. Another risk condition is the primo-infection by T. gondii during pregnancy, which potentially could cause abortion and several congenital malformations in the fetus (Halonen and Weiss, 2013).
As a member of the phylum Apicomplexa, T. gondii has an obligatory intracellular lifestyle (Adl et al., 2007); therefore, invading a host cell, establishing it inside a parasitophorous vacuole (PV) and its later differentiation to tissue cyst are mandatories for the establishment of infection by this parasite. The construction of a perfect intracellular niche by T. gondii requires the secretion of an arsenal of proteins from secretory organelles, such as micronemes, rhoptries and dense granules. These secreted proteins have crucial roles in host cell invasion and later in remodeling the vacuolar environment as well as in interfering with the host cell organization and functions, allowing the parasite to access essential nutrients and defying host cell recognition to become “invisible” (Sinai, 2013; Clough and Frickel, 2017). In this review, we will discuss the main steps involved in the PV construction and cyst differentiation, focusing mainly on the strategies employed in nutrient acquisition and proteins involved in host cell modification.
The Process of Toxoplasma gondii Invasion
The invasion by T. gondii is an active process powered by the parasite gliding motility (Sibley et al., 1998; Frénal et al., 2017) and consists of multiple steps that require the sequential and orchestrated secretion of micronemes, rhoptry, and dense granules (Carruthers and Sibley, 1997). The secretion of micronemes in T. gondii is triggered by the intracellular increase of Ca2+ (Carruthers and Sibley, 1999; Lourido and Moreno, 2015). Microneme proteins (MICs) are secreted at the apical end of T. gondii and are essential for gliding motility and invasion, acting as major recognizers and ligands of the host cell surface and substrates (Carruthers et al., 1999; Soldati et al., 2001; Huynh and Carruthers, 2006). The strong binding of MIC proteins to the host cell surface triggers the apical secretion of rhoptry content into the cytoplasm of host cells by a transient pore (Carruthers and Boothroyd, 2007).
Rhoptries are club-shaped organelles with two different regions that contain a different set of biological roles. While proteins from the rhoptry neck (RONs) act in forming a structure at the surface of the host cell called the moving junction (MJ), the proteins from its bulb (ROPs) act in modifying the PV and modulating the host cell response (Table 1) (Ben Chaabene et al., 2021). The MJ is molecularly composed of rhoptry neck proteins RON2, RON4, RON5, and RON8, and also the microneme protein AMA-1. RON2 is inserted into the host cell membrane and acts as a ligand for AMA1 at the parasite membrane surface. RON4, RON5, and RON8 locate in the host cell cytoplasm and mediate the interaction between the cytoskeleton proteins of the host cell and the cytoplasmic portion of RON2. The MJ forms a juxtaposition between the membranes of the parasite and the host cell and anchors the parasite to the host cell surface as it moves to force the invagination of the host cell membrane, leading then to the formation of the PV during the invasion process (Besteiro et al., 2011; Horta et al., 2020).
At the end of the invasion process, the secretion of the third set of organelles, known as dense granules, starts (Carruthers and Sibley, 1997). Contrasting to the microneme, cytosolic Ca2+ increase negatively regulates dense granule secretion in T. gondii (Katris et al., 2019). Thus, a decrease in cytosolic Ca2+ is important to shift tachyzoite from an invasive to a non-motile state, permitting its intracellular establishment and development (Katris et al., 2019). Interestingly, bradyzoites also show a strong suppression of Ca2+ signaling that restricts parasite egress and maintains the life-long chronic infection (Fu et al., 2021).
Initial steps of the formation of parasitophorous vacuole
The detachment of PV from the host cell plasma membrane was shown to be mediated by the GTPase dynamin (Caldas et al., 2009), which is known for the intracellular release of nascent endocytic vesicles by catalyzing the membrane fission (Williams and Kim, 2014). Intriguing, later studies also found labeling for dynamin inside the parasitophorous vacuole (Caldas et al., 2016). Besides dynamin, a more recent study also showed another mechanism independent of this protein, in which the PV pinch-off would occur mechanically in a process mediated by the tachyzoite twisting motion (Pavlou et al., 2018).
Electrophysiological measurements using a patch-clamp confirmed that the PV membrane (PVM) originates through the invagination of the host cell plasma membrane (Suss-Toby et al., 1996). Thus, until the detachment of the nascent PV from the host cell plasma membrane, PVM lipidic composition is the same as that of the host cell plasma membrane. Accordingly, studies using different lipid probes or lipid targeted labeling confirmed that host cell plasma membrane lipids such as cholesterol, glycolipids, and phospholipids, contained in domains or not, flow freely into the forming PVM (Mordue et al., 1999a; Charron and Sibley, 2002; Coppens and Joiner, 2003).
In contrast to the observed lipidic composition, proteins from the host cell plasma membrane cannot flow freely to the forming PVM, and during the moment of invasion, most proteins from the host cell membrane are selectively prevented from accessing the nascent PVM (Porchet-Hennere and Torpier, 1983; Mordue et al., 1999a; Charron and Sibley, 2004). GPI-anchored proteins, non-multimeric cytosolic leaflet acylated proteins, and some raft-associated transmembrane proteins are internalized from the plasma membrane into the PV, but cytoskeletal linked proteins and multimeric protein complexes, associated or not to raft domains, are barred (Mordue et al., 1999a; Charron and Sibley, 2004). Supporting this finding, studies using freeze-fracture showed a low amount of intramembranous particles at the PVM during and in the early moments after the invasion (Porchet-Hennere and Torpier, 1983; Lemgruber et al., 2008). Rab5 and Rab7 are among the proteins removed from the nascent PVM during the active invasion. These molecules would route the PV towards the endocytic pathway and fusion with lysosomes, causing parasite death (Mordue and Sibley, 1997). Thus, PV is segregated from the endocytic and exocytic pathways of the host cell (Mordue et al., 1999b) and maintains a neutral pH (Sibley et al., 1985).
Remodeling of parasitophorous vacuole after the invasion
Parasitophorous vacuole membrane:
After the end of the invasion, the volume of the PV modifies over time, and from an initial tighten vacuole (Fig. 1A), it enlarges while accommodating new tachyzoites resulting from replication cycles (Figs. 1B–1D) (Mordue et al., 1999b; Lemgruber et al., 2008). The PV enlargement involves the mobilization of lipids from different host cell sources, which are then incorporated into the PVM (Melo and de Souza, 1996, 1997; Charron and Sibley, 2002; Caffaro and Boothroyd, 2011). Accordingly, the composition of PVM intramembranous particles also shows a progressive increase over time and reaches a peak around 6 h post-infection (Porchet-Hennere and Torpier, 1983; Lemgruber et al., 2008). The PVM projections extend into the host cytoplasm and interconnect PVs in the same host cell and located in neighboring cells too (Dubremetz et al., 1993; Rome et al., 2008; Coppens et al., 2006; Lige et al., 2011; Romano et al., 2013). In addition, some PVM projections interact with host organelles, affecting host cell dynamics or getting nutrients from host organelles (Coppens et al., 2006; Romano et al., 2013).
Figure 1: Remodeling of parasitophorous vacuole (PV) after tachyzoite invasion. (A) PV after 2 h of invasion, arrow points to the parasite convolved basal end secreting filamentous nanotubular materials; (B) PV after 4 h of invasion, short arrows point endoplasmic reticulum (ER), large arrows point to PVM invaginations into PV, and arrowheads point the tubular intravacuolar network (IVN); (C) PV after 12 h of invasion, arrowheads point to the tubular IVN; (D) PV after 24 h of invasion, arrowheads point to the tubular IVN connecting the tachyzoite rosette to PVM. Inset of (D) shows pore at the PVM (arrow). (A–C) are transmission electron microscopy images, and (D) a scanning electron microscopy image. HC–host cell; M–mitochondrion.
Most identified PVM proteins are secreted from the dense granules and rhoptries (Tables 1 and 2). While rhoptry proteins decorate the cytoplasmic face of PVM (Bradley and Sibley, 2007), dense granule proteins are more diverse with regards to PVM localization and are found on both cytoplasmic or matrix sides of PVM (Mercier et al., 2005; Mercier and Cesbron-Delauw, 2015). Several of those proteins had their roles already elucidated and will be further addressed herein.
Among the rhoptry proteins with PVM localization and known role (Table 1) are ROP1, ROP2, ROP4, ROP5, ROP7, ROP8, ROP17, ROP18, and ROP54 (Beckers et al., 1994; Bradley and Boothroyd, 2001; El Hajj et al., 2007b; Reese et al., 2014; Kim et al., 2016). Rhoptry bulb ROP1 can be detected at the nascent PVM during parasite invasion and then quickly disappears (Bradley et al., 2002). ROP2, ROP4, ROP5, ROP7, ROP8, and ROP18, are members of the ROP2 family, a group of kinase-related proteins (Beckers et al., 1994; El Hajj et al., 2006). Members of this family of proteins are seen at the nascent PVM during the invasion and persist at this location during the cell infection, acting as virulence factors (see Table 1) (El Hajj et al., 2006, 2007a; Saeij et al., 2006). ROP5 and ROP18 are the major determinants of the differences in the virulence phenotype between T. gondii strains in mice (Shwab et al., 2016) and together with ROP17, disrupt the immunity-related GTPases (IRG) (Etheridge et al., 2014; Reese et al., 2014; Behnke et al., 2015).
Recently, the use of proximity-labeling-based methods allowed the identification of a high amount of GRA proteins, and several of them show PVM localization (Table 2). Secreted GRA proteins present highly diverse roles, such as host cell immune modulation (GRA7, GRA12, GRA15, and GRA60) (Rosowski et al., 2011; Alaganan et al., 2014; Fox et al., 2019; Nyonda et al., 2021), nutrient acquisition (GRA2, GRA7, GRA17, and GRA23) (Coppens et al., 2006; Dou et al., 2014; Gold et al., 2015), translocation of GRA effector proteins to host cytosol (MYR1-4, GRA44, and 45) (Rastogi et al., 2019; Cygan et al., 2020), host organelles–PV association (mitochondrial association factor 1 (MAF1) and GRA3) (Pernas et al., 2014; Deffieu et al., 2019), and chronic infection establishment (GRA4, GRA6, GRA7, GRA8, and GRA14) (Guevara et al., 2019, 2020).
Besides proteins secreted by T. gondii, components of the host endosomal sorting complexes required for transport (ESCRT) complex are also recruited for the cytosolic face of PVM (Guérin et al., 2017; Cygan et al., 2021; Rivera-Cuevas et al., 2021). ESCRT machinery is a conserved membrane remodeling complex that acts in membrane repair and scission, cell cytokinesis, and degradation of membrane proteins (Olmos, 2022). Recruitment of ESCRT machinery is possibly mediated by GRA proteins (GRA14 and GRA64) and would allow T. gondii to access and internalize host cytosolic proteins across PVM (Rivera-Cuevas et al., 2021; Mayoral et al., 2022).
The intravacuolar network (IVN):
Another drastic modification seen in PV after T. gondii invasion is the presence of vesicles, granular material, and the formation of a network of membranous tubules known as the intravacuolar network (IVN) at the PV matrix (Figs. 1B–1D) (Sibley et al., 1995; Mercier et al., 2002; Magno et al., 2005; de Souza and Attias, 2015). Observations by transmission electron microscopy (TEM) showed filamentous nanotubular materials close to the convolved basal end of the parasite a few hours after the end of the invasion (arrow Fig. 1A). These findings suggest that the IVN secretion by T. gondii initiates at the early moments after the invasion (Sibley et al., 1995; Lemgruber et al., 2008; Attias et al., 2019). However, later during infection, the lipidic composition of IVN seems to be mostly mobilized from the host (Caffaro and Boothroyd, 2011). Nanotubular IVN has a bilayer profile and is continuous with the PVM (Sibley et al., 1995). As the infection progresses, membranous tubes enlarge and increase in amount (Fig. 1C), forming an extensive network of variable length that is interconnected by a filamentous material that fills the PV lumen and connects tachyzoites to the PV membrane (Figs. 1B and 1D) (Sibley et al., 1995; Magno et al., 2005; de Souza and Attias, 2015).
The tubular shape of the IVN membrane is believed to involve the association of dense granule proteins GRA2 and GRA6 (Mercier et al., 2002; Travier et al., 2008; Lopez et al., 2015). GRA2 and GRA6 proteins contain amphipathic alpha-helical and hydrophobic alpha-helical domains, respectively, in their structure, allowing their insertion into IVN membranes and shaping their curvature (Travier et al., 2008; Lopez et al., 2015; Bittame et al., 2015). Phosphorylation of GRA2 and GRA6 by a secreted kinase, previously identified as ROP35 (Table 1), also seems to have an important role in the association and stabilization of these proteins in IVN membranes (Beraki et al., 2019). Deletion of GRA2 and disruption of GRA6 genes resulted in a PV devoid of membranous tubular structures (Mercier et al., 2002; Lopez et al., 2015; Rommereim et al., 2016). Other GRAs also associated with IVN tubular structures are GRA4, GRA7, GRA9, GRA12, and GRA41 (Labruyere et al., 1999; Adjogble et al., 2004; Michelin et al., 2009; LaFavers et al., 2017). Deletion of GRA41 caused disorganization of IVN formation, similar to that seen for GRA2, but vacuoles from GRA7-deleted parasites showed a hyper-formation of the IVN membranes, suggesting that both are also important for IVN formation or stabilization (Rommereim et al., 2016; LaFavers et al., 2017).
Within the IVN membrane tubules functions are the organization of daughter cells rosettes resulting from replication cycles, acting in the connection between the parasites and between them and the PVM (Magno et al., 2005; Travier et al., 2008; de Souza and Attias, 2015), the transport of nutrients or translocation of material from the host cell cytosol to PV (Coppens et al., 2006; Dou et al., 2014; Romano et al., 2017), and even to immune modulation by limiting major histocompatibility class I antigen presentation in immune cells (Lopez et al., 2015; Rommereim et al., 2019).
After several replication cycles of tachyzoites, which cause host cellular lysis (Blader et al., 2015), the infection progresses from an acute stage to a chronic phase of the disease, characterized by the presence of tissue cysts that persist for the whole life of infected individuals. The beginning of the chronic phase requires the differentiation of fast-growing tachyzoites to the slow-growing form of bradyzoite. Differentiation to bradyzoite is induced by the pressure of the host immune system and other stress factors. Bradyzoites modify the PV and construct a wall beneath PVM, forming intracellular tissue cysts (Augusto et al., 2021). The cyst wall is a thick and electron-dense structure, which isolates the bradyzoites from the host cell cytoplasm and the surrounding environment. Bradyzoites duplicate slowly within tissue cysts and are predominantly found in the brain, retina, and muscles. Cysts are not static forms of T. gondii but dynamic structures that can exchange molecules with the host cell, rupture, and release parasites. The viability of parasites within the cysts is possible due to the permeability of the cyst wall to nutrients that can pass from the host cell cytoplasm into the cyst (Paredes-Santos et al., 2018, 2019).
Our group described the cyst wall with an irregular and rough surface by scanning electron microscopy. The cyst is enclosed by a cyst wall which is a remnant of the PVM, which becomes convoluted and connected to a great amount of fibrillar and electron-dense material in the internal face of the PVM (Lemgruber et al., 2011). In addition, the cyst wall exhibits small vesicles and membranous tubules immersed in the fibrillar network (Lemgruber et al., 2011; Tomita et al., 2013). T. gondii cyst wall consists of two layers–the outermost thin layer, composed of compact material, and an innermost, thicker layer of a sponge-like appearance that faces the cyst matrix. Antibodies for GRA1, GRA3, and GRA7 stain both the convoluted cyst membrane and the cyst wall filamentous components. A great number of vesicles of about 67 nm in diameter are present within the cyst wall, likely transporting wall components.
Using in vitro model of cystogenesis, it was possible to verify that the deposition of cyst wall components occurs before the full conversion of tachyzoites to bradyzoites. Stage-specific proteins are expressed at different steps throughout the process of conversion of tachyzoites to bradyzoites. The cyst wall is abundant in highly glycosylated proteins with terminal residues of N-acetyl-galactosamine and N-acetyl-glucosamine that are recognized by the lectins Dolichos biflorus agglutinin (DBA) and wheat germ agglutinin (WGA) respectively (Derouin et al., 1981). The bradyzoite proteins bradyzoite antigens (BAG1) and cyst wall protein (CST1) are found at the beginning of the in vitro differentiation. Whereas lactate dehydrogenase (LDH2) and surface proteins, such as SAG1-related sequences (SRS9), appear later in the differentiation to bradyzoites (Yang and Parmley, 1997; Kim and Boothroyd, 2005; Paredes-Santos et al., 2018).
The CST1 is the major protein in the cyst wall; the purified protein is a 250-kDa protein rich in mucins in the N-terminal region that present a high affinity for the DBA lectin. CST1 possibly has a structural role in the support of the cyst wall, and parasites knockouts for this protein, or that do not express the mucin-rich region, form fragile cysts in vivo (Zhang et al., 2001; Tomita et al., 2013). The inhibition of an initial precursor of the parasitic o-glycosylation pathway reduced the persistence of infection in vivo, and cysts were not recognized by DBA (Caffaro et al., 2013), indicating the glycosylation is essential for the addressing and assembling of proteins of the wall. CST2, another cyst wall protein, was found in the cyst matrix (Table 2) and was related to the virulence and the establishment of the chronic phase of T. gondii infection. Mice infected with CST2-KO parasites showed inconspicuous cyst burden (Tu et al., 2019). Mice infected with knockouts for GRA4 and GRA6 also presented with fewer cysts (Fox et al., 2011).
The intracyst network (ICN) is another component of the cyst. ICN consists of a diffuse material between the bradyzoites and an intricate network of tubules similar but shorter, denser, and with vesicles of a different diameter than those present in the IVN. Electron tomography and thin sections of TEM showed that these vesicles buds from the cell body and the posterior pole of bradyzoites forming the tubule-vesicular network of the ICN, both in vivo and in vitro (Lemgruber et al., 2011; Paredes-Santos et al., 2018). Several proteins secreted by the parasite have already been described as components of the cyst matrix and ICN (Table 2).
Considering the origin of the cyst wall, our group studied an in vitro model of cystogenesis by a series of electron tomography 3-D reconstructions and brain-isolated cysts by several TEM micrographs. After these studies, our group proposed that PVM remained intact as it transitioned into the cyst membrane by the accumulation of parasite molecules derived from vesicles secreted by intracystic bradyzoites (Lemgruber et al., 2011; Paredes-Santos et al., 2018). Later, Guevara and Col showed that GRA5 and GRA7 are localized at the PVM and were visible at the cyst periphery from 6 h after differentiation. Besides, the deletion of PVM- and IVN-associated GRA proteins, such as GRA3, GRA5, GRA7, GRA8, and GRA14, showed the importance of these molecules to support the correct accumulation of cyst wall proteins to cyst periphery. These results indicated that the PVM-localized GRAs assist the accumulation of cyst wall components in the cyst wall periphery (Guevara et al., 2020).
A study comparing the transcriptome of tachyzoites and bradyzoites from cell culture in vitro and brain cysts from infected mice identified two bradyzoite-specific proteins localized in the cyst wall: one with a microneme adhesive repeat (MAR)-like adhesive domain- the (Microneme Adhesive Repeat domain-containing protein 4 (MCP4), and the bradyzoite pseudo-kinase BPK-1 (Buchholz et al., 2011). BPK-1 is essential for resistance to the active pepsin enzyme present in the low pH of gastric juice, which is the first barrier faced by the parasite after the oral ingestion of tissue cysts (Buchholz et al., 2013). The resistance to gastric juice is crucial for the transmission of T. gondii after consuming raw or undercooked meat from chronically infected animals (ingestion of tissue cysts).
Nutrient acquisition through parasitophorous vacuole membrane and cyst wall
For an intracellular parasite, residing in a membrane-bounded intracellular compartment could mean an advantage because this physical barrier protects it from being sensed by the host cell but also present the disadvantage of the lack of free access to solutes from the host cell cytosol. In the case of T. gondii, this is even more complicated, as the PV is also excluded from the host endosomal system. However, through evolution, several mechanisms allowed the acquisition of essential molecules from the host.
Pores at the PVM (Fig. 1D inset) (de Souza and Attias, 2015) make PV permeable to any molecule up to 1.3 kDa (Schwab et al., 1994), then allowing the passive transport of small molecules or ions from the host cell cytosol to the PV matrix. Thus, T. gondii can access metabolites such as glucose, iron, essential amino acids, purines, lipids, and inorganic ions, which are essential for its growth (Blume and Seeber, 2018). The molecular basis of the PVM pore consists of dense granule proteins GRA17 and GRA23 (Gold et al., 2015). GRA17 is related to the putative Plasmodium translocon protein EXP2, a protein-conducting pore of the translocon for exported proteins (PTEX) and a permeable channel that facilitates the transport of nutrients into the Plasmodium PV (Koning-Ward et al., 2009; Garten et al., 2018). Interestingly, homologs for those proteins were only found in Apicomplexa that replicates inside a PV (Gold et al., 2015). GRA17 and GRA23 act synergistically in the permeability of PV in tachyzoites (Gold et al., 2015). Tachyzoites presenting deleted GRA17 presented swollen bubble vacuoles suggesting the possible role of GRA17 pores in the secretion of toxic metabolic byproducts produced by parasites into the host cell (Gold et al., 2015). GRA17 also acts in the permeability cyst wall and the acquisition of molecules by bradyzoites through it (Paredes-Santos et al., 2019). Permeability studies with tissue cysts isolated from mice brain showed that molecules up to 10 kDa could cross the cyst wall (Guimarães et al., 2007; Lemgruber et al., 2011).
Even apart from host endosomal and secretory pathways, T. gondii can mobilize and internalize in the vacuolar space host endocytic organelles, multivesicular bodies, lipid droplets (LD), and a broad range of Rab vesicles sequestered from secretory and endosomal pathways (Coppens et al., 2006; Romano et al., 2013, 2017; Dou et al., 2014; Nolan et al., 2017). Vesicle sequestration is a route for acquiring lipids and proteins from the host cell (Coppens et al., 2006; Dou et al., 2014; Romano et al., 2017). Notably, T. gondii is unable to synthesize any kind of sterol and strictly depends on the host to obtain this lipid. Cholesterol is acquired from the host low-density lipoprotein endocytosis pathway and not from the endogenous biosynthetic pathway (Coppens et al., 2000). Internalization of endocytosed cholesterol occurs through the sequestration of host endo-lysosomes vesicles through microtubular conduits of PVM invaginations mediated by microtubules, named Host Organelle-Sequestering Tubulo-structures (HOST). HOST are stabilized by the GRA7 protein, which forms a coat and would act like a garrote sequestering the host endocytic vesicles (Coppens et al., 2006). Indeed, IVN tubules attached to PVM are also important in the interception of host Rab vesicles (Romano et al., 2017). Vacuoles with parasites deleted for GRA2 and GRA6 proteins, i.e., with a non-functional IVN, scavenge fewer host-derived materials like host Rab vesicles, endosomes, LD, and host cytosolic proteins (Dou et al., 2014; Romano et al., 2017). Although the mechanisms of nutrient acquisition through host organelle mobilization by tachyzoites are broadly known, it has been only partially identified in bradyzoite. During cystogenesis and later, tissue cysts maintain a close association with endoplasmic reticulum (ER) and also showed an accumulation of lysosomes around their vicinity (Paredes-Santos et al., 2018), but the sequestration of these organelles inside the tissue cyst has not been shown yet.
Besides the role of LD in the acquisition of lipids, it also appears to participate in controlling the infection by the parasite. The infection of skeletal muscle primary culture with tachyzoites from RH strain enhanced the LD number and the levels of IL-12, IFN-γ, and inflammatory indicators prostaglandin E2 (PGE2) and cyclooxygenase. These LDs were found close to the sarcoplasmic reticulum and disposed near the PV (Fig. 2E), indicating that it could be a source of lipids and nutrients for the parasite development, while the inflammatory profile identified could be related to the establishment and maintenance of chronic phase of the T. gondii infection in the muscle (Gomes et al., 2014). Studies investigating the interaction of peritoneal macrophages with tachyzoites when cultured with mouse serum showed higher numbers of LDs than those cultured in fetal bovine serum. The LDs could reduce the microbicidal activity of macrophages against T. gondii by increasing the PGE2 while diminishing nitric oxide production (Mota et al., 2014).
Figure 2: Recruitment of host cell structures and organelles to the parasitophorous vacuole (PV) periphery. (A and inset). PV with tachyzoites showing host cell microtubules encircling its periphery (arrows); (B and inset). In vitro intracellular cyst showing host cell microtubules (arrows) close to cyst wall (CW) periphery. Bradyzoites present dense rhoptries (Rp), posterior nucleus (N) and amylopectin granules (arrowheads); (C) recruitment of actin filaments (arrow) during the invasion of host cell by tachyzoites (encircled by dashed line); (D)–PV membrane showing association to mitochondrion (M); (E) PVM showing association to host cell Golgi complex (GC; arrows), mitochondria (M) and Lipid droplet (LD; arrowheads). (A–B) and (D–E), Transmission electron microscopy images. (C) is a fluorescence image of a host cell labeled with phalloidin. Bars A–E –1 µm. Bars B inset 0.5 µm.
Modifications in host cell organization and gene expression
Nevertheless, host cell components reorganize following the first steps of T. gondii invasion. This reorganization includes the recruitment near the PV host organelles and structures like the nucleus, the centrosome (Coppens et al., 2006; Wang et al., 2010), ER (Melo and de Souza, 1997), mitochondria (de Melo et al., 1992; Sinai et al., 1997), the Golgi complex (de Melo and de Souza, 1996; Coppens et al., 2006; Deffieu et al., 2019), microtubules, and intermediate filaments to the vicinity of PV (Melo et al., 2001; Coppens et al., 2006).
T. gondii induces major changes in host cell cytoskeleton organization. After the invasion, the host cell centrosome is recruited away from the nuclear membrane and allocated close to the PVM (Coppens et al., 2006; Walker et al., 2008; Wang et al., 2010; Romano et al., 2013). The centrosome is usually associated with the nucleus and has a major role in organizing the microtubule cytoskeleton in animal cells, which could explain in part the mechanism that T. gondii can remodel host microtubules. In the first few minutes post-invasion, the PV is enclosed by microtubules (Melo et al., 2001; Walker et al., 2008), and this configuration remains at later times of infection with tachyzoites (Fig. 2A) when the PV localizes in the perinuclear region. However, repositioning of the centrosome from the nucleus to the vicinity of PV occurs only 24 h post-invasion (Romano and Coppens, 2013; Cardoso et al., 2014). The presence of microtubule at or near the moving junction of T. gondii before invasion appears to speed up the process of entry of the parasite into the cell (Sweeney et al., 2010). After the end of the invasion process, microtubules cluster around PV and form a basket-like organization, which persists even during chronic infection (Fig. 2B) (Walker et al., 2008; Paredes-Santos et al., 2018). The proximity of microtubules to PV later during infection would allow the acquisition of several important molecules and nutrients, facilitate the arrival of the parasite molecules into the host organelles, and provide mechanical support for tachyzoites to divide inside the PV (Cardoso et al., 2016). The host-signaling pathway that was first described as crucial in the reorganization of the cytoskeleton of cells after invasion by T. gondii was the host mTORC2-Akt signaling. This pathway regulates the localization of the host centrosome, the organization of microtubules, and the distribution of mitochondria and lysosomes around the PV (Wang et al., 2010).
The recruitment of actin for the entry site is also needed for the invasion of the parasite into the host cell. A host cell-derived ring-shaped F-actin structure is formed at the MJ (Fig. 2C) and disappears a few minutes after tachyzoite entrance (Frénal and Soldati-Favre, 2009). Actin reorganization at nascent PVM during host entrance involves host cell ADP-ribosylation factor-6 (ARF6), a small GTPase that acts in the actin cytoskeleton rearrangements at the plasma membrane (da Silva et al., 2009), and RhoA and Rac1 GTPases (Na et al., 2013), which are known regulators of the organization and dynamics of the actin cytoskeleton (Sit and Manser, 2011). Early studies also showed that the host intermediate filaments are disposed around the PVM and are crucial to the PV location near the nucleus and the PV shape maintenance (Halonen and Weidner, 1994). Intermediate filaments also seem to regulate the intracellular proliferation and the establishment of chronic infection of T. gondii. In human brain microvessel endothelial cells knockout for vimentin, the T. gondii proliferation was increased in comparison to wild cells. Similarly, in the mouse tissues, the levels of vimentin amount also seem to affect the development of the infection by T. gondii, and the low level of this filament in the brain could be associated with the tropism of the parasite for this tissue site, where the infection by cysts can develop (He et al., 2017).
T. gondii PVM also exhibits a remarkable association with host organelles such as mitochondria (Fig. 2D), ER (Fig. 1B), and Golgi complex (Fig. 2E) (Jones and Hirsch, 1972; Melo and de Souza, 1997). The association with ER would allow the increase in the size of the PVM, necessary during the parasite replication (Melo and de Souza, 1997); and the antigen-cross presentation in dendritic cells infected by T. gondii (Goldszmid et al., 2009). The interaction of ER and PVM would be mediated by the dense granule protein GRA3 of T. gondii (Kim et al., 2008). The PV preferentially localizes close to the host Golgi within the first few hours of infection (2 hpi) (Romano et al., 2013) and this localization persists during the chronic infection (Paredes-Santos et al., 2018). Proximity with Golgi is a privileged localization, favoring the parasite to acquire nutrients derived from its apparatus, such as the lipid ceramide, and also through the sequestration of vesicles from host cell of endocytic and exocytic pathways (de Melo and de Souza, 1996; Dou et al., 2014; Romano et al., 2017). The interaction of PV with Golgi is directly mediated by TgGRA3, which is involved in the formation of tubules and the entry of host Golgi material into the PV (Deffieu et al., 2019). Mitochondria recruitment to PVM is strain-specific and involves the secreted factor MAF1b (Pernas et al., 2014; Blank et al., 2018). Host mitochondria-PV association occurs at high rates in type I and type III strains but is rarely seen in type II strains (Pernas et al., 2014). Mitochondrial-PV association would be mediated by the interaction between MAF1b and host mitochondrial outer membrane proteins, translocase of outer mitochondrial membrane 70, and heat shock protein family A9 (Kelly et al., 2017; Blank et al., 2021). Recent work also identified ROP39 as a new recruitment factor for mitochondria (Fukumoto et al., 2021). The association of PV with host mitochondria would allow T. gondii to access host cell metabolites (Crawford et al., 2006; Fu et al., 2018) and interfere with the host immune response enhancing parasite survival (Pernas et al., 2014). Transcriptomic data showed that host mitochondrial association to PV of T. gondii from type I virulent strain affected gene expression profiling of infected cells, which showed alteration in the expression of genes related to host mitochondrial dysfunction. Morphological analysis shows the fast recruitment of mitochondria around the PV, as known, and the presence of fragmented host mitochondria, a profile not associated with cellular apoptosis (Syn et al., 2017).
Effector proteins secreted from rhoptries and dense granules can interact with host cell signaling and transcription, modulating gene expression, immune response, cell division, apoptosis, and metabolism (Sanchez and Besteiro, 2021). T. gondii infection induces c-Myc, a multifunctional transcription factor that plays a central role in the growth and division of animal cells (Dang, 2012; Franco et al., 2014). The regulation of c-Myc is dependent on MYR (Myc regulatory genes) proteins (Cygan et al., 2020), which act in the translocation of several GRAs effectors (GRA16, GRA18, GRA24, HCE1/TEEGR, TgNSM, and TgIST) across the PVM (Table 2) (Naor et al., 2018; Rastogi et al., 2019). Although T. gondii-mediated regulation of c-Myc is not known much, it possibly influences the host cell cycle (Brunet et al., 2008; Molestina et al., 2008; Velásquez et al., 2019). Within translocated GRA proteins, TgGRA16 is important for the accumulation of host c-Myc (Panas and Boothroyd, 2020). However, the deletion of GRA16 did not entirely abolish the c-Myc response, but MYR1 deletion caused a 97% reduction, suggesting that other(s) MYR complex dependent-effector(s) could upregulate the expression of c-Myc mRNA (Bougdour et al., 2013; Panas and Boothroyd, 2020). GRA16 would also impact the cell cycle through p53 modulation, allowing the host cell to survive under stress conditions. Parasites from GRA16-deficient strains presented attenuated virulence, indicating the importance of these host alterations in pathogenesis (Bougdour et al., 2013). Other T. gondii effectors reported to impact the host cell cycle are GRA24 and ROP38, both targeting p38 mitogen-activated protein (MAP) kinase (Peixoto et al., 2010; Braun et al., 2013), and HCE1/TEEGR, which alters the expression of cyclin E (Panas et al., 2019b).
Mechanisms of intracellular immune effectors evasion
INF-γ is essential for controlling both acute and chronic infection (Gazzinelli et al., 1994; Yap et al., 2000). Secretion of IFN-γ is stimulated in response to the IL-12 secretion, followed by the recognition of T. gondii by dendritic cells, macrophages, and monocytes (Sher et al., 2003; Tosh et al., 2016). IFN-γ activates the Janus kinase (JAK) signal transducer and activator of the transcription 1 (STAT1) pathway, which induces the expression of classical interferon-stimulated genes (ISGs), crucial for the establishment of a Th1-response (Gazzinelli et al., 1994), and to restrict the intracellular infection in hematopoietic and non-hematopoietic cells (Yap and Sher, 1999a; Ivashkiv, 2018). The intracellular mechanisms involved in controlling T. gondii proliferation include the production of reactive oxygen species, degradation of tryptophan through the expression of indoleamine 2,3-dioxygenase, and the production of nitric oxide through induction of nitric oxide synthase (Yap and Sher, 1999b). IFN-γ also leads to the expression of IRGs (essential for the control of infection in mice) and guanylate-binding proteins (GBPs) (Sasai and Yamamoto, 2019). Thus, through evolution, the surviving strains were those that evolved several secretory factors directed to disrupt the host cell-autonomous immune mediators and modulate the transcription of immune response genes (Tables 1 and 2), especially those induced by the IFN-γ/JAK/STAT1 pathway (Hakimi et al., 2017).
As mentioned previously, the escape from IRG is a determinant of the differences in the virulence seen between T. gondii strains in mice. IRG proteins are a large family of IFN-inducible proteins and are essential for resistance to T. gondii infection in mice. IRGs accumulate around PVM and lead to its disruption and consequent parasite death (Taylor, 2007; Zhao et al., 2009). The importance of IRG in the innate immune defense of mice against T. gondii is evident by the high amount of polymorphic virulence factors directed to this system. Within those factors are rhoptry proteins ROP2, ROP4, ROP5, ROP8, ROP17, and ROP18 and dense granule proteins GRA7 and GRA60 (Tables 1 and 2). ROP5 is a highly polymorphic pseudokinase (Reese et al., 2014). In strains virulent in mice, ROP5 acts as an allosteric inhibitor of IRG, preventing its oligomerization to PVM and facilitating the phosphorylation and permanent inhibition of IRG monomers by ROP18 kinase (Steinfeldt et al., 2010; Fleckenstein et al., 2012). ROP17 kinase also phosphorylates and inactivates oligomers of IRG, acting synergistically with ROP18 to prevent IRG deposition at PVM (Etheridge et al., 2014). ROP18 also seems to play an important role in the evasion of GBP recruitment to PVM (Virreira Winter et al., 2011).
Infection by T. gondii also induces a major change in the expression of immune-related genes (Kim et al., 2007), and the modulation of response is dependent on the type of strain. Several virulent secretory effectors are already well characterized in modifying host cell transcription targeting the decrease in the intracellular immune response to infection; within these: ROP16, ROP38, GRA12, GRA24, TgIST, and TgNSM (Tables 1 and 2). One well-characterized T. gondii effector known to modify host cell transcription is ROP16, a kinase that in type I/III strains directly phosphorylates the transcription factors STAT3 and STAT6 downstream of IL10/JAK and IL4/JAK cascade, respectively (Yamamoto et al., 2009; Ong et al., 2010; Butcher et al., 2011). Activation of STAT3 and STAT6 leads to their translocation to the host nucleus. In type I strains, the activation of STAT3 and STAT6 promotes the downregulation of proinflammatory cytokine signaling and induces the infected macrophages to an alternatively (M2) activated phenotype, respectively (Denkers et al., 2012). Different from M1-activated macrophages, M2-polarization favors the expression of arginase-1 instead of iNOS, which decreases the synthesis of nitric oxide, an important antimicrobial effector for the control of T. gondii proliferation (Martinez and Gordon, 2014). In type III strains, the activation of STAT6 by ROP16 suppresses the IFN-γ-independent production of reactive oxygen species by human and mouse cells (Kochanowsky et al., 2021).
Two secreted dense granule proteins (TgIST and TgNSM) were recently identified as responsible for decreasing the expression of ISG in host cells. TgIST (T. gondii inhibitor of STAT1 transcriptional activity) is secreted both by tachyzoites and bradyzoites (Olias et al., 2016; Gay et al., 2016; Mayoral et al., 2020). TgIST is translocated through PVM and cyst wall by MYR1 and targets the host cell nucleus, which sequesters STAT1 and recruits Mi-2 nucleosome remodeling and deacetylase (Mi-2/NuRD) complex, resulting in the alteration of chromatin and blocking of the transcription of ISGs (Gay et al., 2016). In human cells, the resistance to parasite killing mediated by TgIST seems to be mainly due to a decrease in IDO1 activity (Bando et al., 2018). TgIST also blocks type I INF signaling by inhibiting STAT1/STAT2-mediated transcription in infected cells (Matta et al., 2019). TgNSM is secreted by bradyzoites and targets the nuclear receptor corepressor/silencing mediator of retinoic acid and thyroid hormone receptor (NCoR/SMRT) complex, a repressor for several transcription factors, including genes involved in cell death. Together with TgIST, TgNSM prevents host cell necroptotic death, protecting the latent intracellular niche of the parasite (Rosenberg and Sibley, 2021).
Different from protozoans that have their PV fused with host cell lysosomes, or multiply in the host cell cytosol, T. gondii creates its ambiance by contacting only the host cell surface, which constitutes the walls of the tachyzoite's room, for example. Because of this, it is plausible to infer that the PV is like a host cell pocket resulting from the invagination of the host cell plasma membrane. Since the concept of an obligatory intracellular parasite is largely defined by the necessity of the host cell intracellular ambiance for the replication of the parasite, this unique parasite can challenge this convention.
In recent years several studies provided a great abundance of information about the PV-host cell interface and the parasite-secreted proteins, especially the dense granules proteins, involved in the construction of IVN in the PV matrix, the interaction of PV-host cell organelles, nutrient acquisition, host cell reorganization, and modulation of host cell gene expression. Nowadays, it is clear that T. gondii resides in a permeable compartment and can access solutes from host cell cytosol. Indeed, although T. gondii VP does not fuse with the endolysosomal system, this parasite evolved mechanisms to mobilize lysosomes and endosomes to the vicinity and inside PV, allowing the parasite to acquire essential molecules, such as cholesterol. The presence of a translocon system at PVM also shows that the success of the T. gondii intracellular survival requires the continuous secretion of GRA effectors directed to the host cell nucleus and cytoplasm, where genes related to cell cycle, metabolism, and immune response, for example, are modulated.
While there is extensive information about tachyzoite PV, the knowledge about the biology of bradyzoite tissue cysts and their interaction with the host cell is scarce but has begun to be elucidated. Recent findings support that bradyzoites are not entirely dormant cells. Such as the PVM, the tissue cyst also presents pores and the MYR translocon system. The molecular elucidation of these pores at the cyst wall and their role in the establishment of chronic infection in vivo support that small solutes can freely diffuse to the cyst matrix and that bradyzoites are metabolically active, although in a reduced way when compared to tachyzoites. Indeed, recent studies showed that bradyzoites also secrete effector proteins targeted to host cell cytoplasm through the MYR translocon and modulate its immune response, especially ISG. This demonstrates that the evasion of bradyzoites/tissue cysts from the host immune response is not due to the presence of the cyst wall, which would act as a mechanical barrier protecting dormant bradyzoites from being detected but requires the parasite to remain active to maintain the status quo of chronic infection. Future studies focused on better understanding how bradyzoites acquire nutrients and evade the immune system of the host cell will open new opportunities for the development of drugs against the chronic phase of toxoplasmosis, which so far has no cure.
Acknowledgement: Images presented in the figures were acquired in the Unidade de Microscopia Avançada at Centro Nacional de Biologia Estrutural e Bioimagem, UFRJ.
Funding Statement: The authors received no specific funding for this study.
Author Contributions: Study conception and design: EMD; data collection: JAP, RCV, LAC, ESMD; draft manuscript preparation: JAP, RCV, LAC, ESMD. All authors reviewed the results and approved the final version of the manuscript.
Availability of Data and Materials: Data sharing is not applicable to this article as no datasets were generated or analyzed during the current study.
Ethics Approval: Not applicable.
Conflicts of Interest: The authors declare that they have no conflicts of interest to report regarding the present study.
References
Adjogble KDZ, Mercier C, Dubremetz JF, Hucke C, MacKenzie CR et al. (2004). GRA9, a new Toxoplasma gondii dense granule protein associated with the intravacuolar network of tubular membranes. International Journal for Parasitology 34: 1255–1264. https://doi.org/10.1080/10635150701494127 [Google Scholar] [CrossRef]
Adl SM, Leander BS, Simpson AGB, Archibald JM, Anderson OR et al. (2007). Diversity, nomenclature, and taxonomy of protists. Systematic Biology 56: 684–689. https://doi.org/10.1080/10635150701494127 [Google Scholar] [PubMed] [CrossRef]
Ahn HJ, Kim S, Nam HW (2007). Nucleolar translocalization of GRA10 of Toxoplasma gondii transfectionally expressed in HeLa cells. The Korean Journal of Parasitology 45: 165–174. https://doi.org/10.3347/KJP.2007.45.3.165 [Google Scholar] [PubMed] [CrossRef]
Alaganan A, Fentress SJ, Tang K, Wang Q, Sibley LD (2014). Toxoplasma GRA7 effector increases turnover of immunity-related GTPases and contributes to acute virulence in the mouse. Proceedings of the National Academy of Sciences of the United States of America 111: 1126–1131. https://doi.org/10.1073/PNAS.1313501111 [Google Scholar] [PubMed] [CrossRef]
Attias M, Miranda K, de Souza W (2019). Development and fate of the residual body of Toxoplasma gondii. Experimental Parasitology 196: 1–11. https://doi.org/10.1016/j.exppara.2018.11.004 [Google Scholar] [PubMed] [CrossRef]
Attias M, Teixeira DE, Benchimol M, Vommaro RC, Crepaldi PH et al. (2020). The life-cycle of Toxoplasma gondii reviewed using animations. Parasites and Vectors 13. https://doi.org/10.1186/s13071-020-04445-z [Google Scholar] [PubMed] [CrossRef]
Augusto L, Wek RC, Sullivan WJ (2021). Host sensing and signal transduction during Toxoplasma stage conversion. Molecular Microbiology 115: 839–848. https://doi.org/10.1111/MMI.14634 [Google Scholar] [PubMed] [CrossRef]
Bai MJ, Wang JL, Elsheikha HM, Liang QL, Chen K et al. (2018). Functional characterization of dense granule proteins in Toxoplasma gondii RH strain using CRISPR-Cas9 system. Frontiers in Cellular and Infection Microbiology 8: 300. https://doi.org/10.3389/FCIMB.2018.00300 [Google Scholar] [PubMed] [CrossRef]
Bando H, Sakaguchi N, Lee Y, Pradipta A, Ma JS et al. (2018). Toxoplasma effector TgIST targets host IDO1 to antagonize the IFN-γ-induced anti-parasitic response in human cells. Frontiers in Immunology 9: 2073. https://doi.org/10.3389/fimmu.2018.02073 [Google Scholar] [PubMed] [CrossRef]
Beckers CJM, Dubremetz JF, Mercereau-Puijalon O, Joiner KA (1994). The Toxoplasma gondii rhoptry protein ROP 2 is inserted into the parasitophorous vacuole membrane, surrounding the intracellular parasite, and is exposed to the host cell cytoplasm. Journal of Cell Biology 127: 947–961. https://doi.org/10.1083/jcb.127.4.947 [Google Scholar] [PubMed] [CrossRef]
Behnke MS, Khan A, Lauron EJ, Jimah JR, Wang Q et al. (2015). Rhoptry proteins ROP5 and ROP18 are major murine virulence factors in genetically divergent South American strains of Toxoplasma gondii. PLoS Genetics 11: e1005434. https://doi.org/10.1371/JOURNAL.PGEN.1005434 [Google Scholar] [PubMed] [CrossRef]
Ben Chaabene R, Lentini G, Soldati-Favre D (2021). Biogenesis and discharge of the rhoptries: Key organelles for entry and hijack of host cells by the Apicomplexa. Molecular Microbiology 115: 453–465. https://doi.org/10.1111/MMI.14674 [Google Scholar] [PubMed] [CrossRef]
Beraki T, Hu X, Broncel M, Young JC, O’Shaughnessy WJ et al. (2019). Divergent kinase regulates membrane ultrastructure of the Toxoplasma parasitophorous vacuole. Proceedings of the National Academy of Sciences of the United States of America 116: 6361–6370. https://doi.org/10.1073/PNAS.1816161116 [Google Scholar] [PubMed] [CrossRef]
Bermudes D, Dubremetz JF, Achbarou A, Joiner KA (1994). Cloning of a cDNA encoding the dense granule protein GRA3 from Toxoplasma gondii. Molecular Biochemical Parasitology 68: 247–257. https://doi.org/10.1016/0166-6851(94)90169-4 [Google Scholar] [PubMed] [CrossRef]
Besteiro S, Dubremetz JF, Lebrun M (2011). The moving junction of apicomplexan parasites: A key structure for invasion. Cellular Microbiology 13: 797–805. https://doi.org/10.1111/j.1462-5822.2011.01597.x [Google Scholar] [PubMed] [CrossRef]
Bittame A, Effantin G, Pètre G, Ruffiot P, Travier L et al. (2015). Toxoplasma gondii: Biochemical and biophysical characterization of recombinant soluble dense granule proteins GRA2 and GRA6. Biochemical and Biophysical Research Communications 459: 107–112. https://doi.org/10.1016/j.bbrc.2015.02.078 [Google Scholar] [PubMed] [CrossRef]
Blader IJ, Coleman BI, Chen CT, Gubbels MJ (2015). Lytic cycle of Toxoplasma gondii: 15 years later. Annual Review of Microbiology 69: 463–485. https://doi.org/10.1146/ANNUREV-MICRO-091014-104100 [Google Scholar] [PubMed] [CrossRef]
Blank ML, Parker ML, Ramaswamy R, Powell CJ, English ED et al. (2018). A Toxoplasma gondii locus required for the direct manipulation of host mitochondria has maintained multiple ancestral functions. Molecular Microbiology 108: 519–535. https://doi.org/10.1111/MMI.13947 [Google Scholar] [PubMed] [CrossRef]
Blank ML, Xia J, Morcos MM, Sun M, Cantrell PS et al. (2021). Toxoplasma gondii association with host mitochondria requires key mitochondrial protein import machinery. Proceedings of the National Academy of Sciences of the United States of America 118: e2013336118. [Google Scholar] [PubMed]
Blume M, Seeber F (2018). Metabolic interactions between Toxoplasma gondii and its host. F1000Research 7. [Google Scholar]
Bougdour A, Durandau E, Brenier-Pinchart MP, Ortet P, Barakat M, et al. (2013). Host cell subversion by Toxoplasma GRA16, an exported dense granule protein that targets the host cell nucleus and alters gene expression. Cell Host & Microbe 13: 489–500. DOI 10.1016/J.CHOM.2013.03.002 [Google Scholar] [PubMed] [CrossRef]
Bradley PJ, Boothroyd JC (2001). The pro region of Toxoplasma ROP1 is a rhoptry-targeting signal. International Journal of Parasitology 31: 1177–1186. https://doi.org/10.1016/S0020-7519(01)00242-9 [Google Scholar] [PubMed] [CrossRef]
Bradley PJ, Hsieh CL, Boothroyd JC (2002). Unprocessed Toxoplasma ROP1 is effectively targeted and secreted into the nascent parasitophorous vacuole. Molecular Biochemical Parasitology 125: 189–193. https://doi.org/10.1016/S0166-6851(02)00162-7 [Google Scholar] [PubMed] [CrossRef]
Bradley PJ, Sibley LD (2007). Rhoptries: An arsenal of secreted virulence factors. Current Opinion in Microbiology 10: 582–587. https://doi.org/10.1016/J.MIB.2007.09.013 [Google Scholar] [PubMed] [CrossRef]
Bradley PJ, Ward C, Cheng SJ, Alexander DL, Coller S et al. (2005). Proteomic analysis of rhoptry organelles reveals many novel constituents for host-parasite interactions in Toxoplasma gondii. The Journal of Biological Chemistry 280: 34245–34258. https://doi.org/10.1074/JBC.M504158200 [Google Scholar] [PubMed] [CrossRef]
Braun L, Brenier-Pinchart MP, Hammoudi PM, Cannella D, Kieffer-Jaquinod S et al. (2019). The Toxoplasma effector TEEGR promotes parasite persistence by modulating NF-κB signalling via EZH2. Nature Microbiology 4: 1208–1220. DOI 10.1038/s41564-019-0431-8 [Google Scholar] [PubMed] [CrossRef]
Braun L, Brenier-Pinchart MP, Yogavel M, Curt-Varesano A, Curt-Bertini RL et al. (2013). A Toxoplasma dense granule protein, GRA24, modulates the early immune response to infection by promoting a direct and sustained host p38 MAPK activation. Journal of Experimental Medicine 210: 2071–2086. https://doi.org/10.1084/jem.20130103 [Google Scholar] [PubMed] [CrossRef]
Brunet J, Pfaff AW, Abidi A, Unoki M, Nakamura Y et al. (2008). Toxoplasma gondii exploits UHRF1 and induces host cell cycle arrest at G2 to enable its proliferation. Cellular Microbiology 10: 908–920. https://doi.org/10.1111/J.1462-5822.2007.01093.X [Google Scholar] [PubMed] [CrossRef]
Buchholz KR, Bowyer PW, Boothroyd JC (2013). Bradyzoite pseudokinase 1 is crucial for efficient oral infectivity of the Toxoplasma gondii tissue cyst. Eukaryotic Cell 12: 399–410. https://doi.org/10.1128/EC.00343-12 [Google Scholar] [PubMed] [CrossRef]
Buchholz KR, Fritz HM, Chen X, Durbin-Johnson B, Rocke DM et al. (2011). Identification of tissue cyst wall components by transcriptome analysis of in vivo and in vitro Toxoplasma gondii bradyzoites. Eukaryotic Cell 10: 1637–1647. https://doi.org/10.1128/EC.05182-11 [Google Scholar] [PubMed] [CrossRef]
Butcher BA, Fox BA, Rommereim LM, Kim SG, Maurer KJ et al. (2011). Toxoplasma gondii rhoptry kinase ROP16 activates STAT3 and STAT6 resulting in cytokine inhibition and arginase-1-dependent growth control. PLoS Pathogens 7: e1002236. https://doi.org/10.1371/JOURNAL.PPAT.1002236 [Google Scholar] [PubMed] [CrossRef]
Butterworth S, Torelli F, Lockyer EJ, Wagener J, Song OR et al. (2022). Toxoplasma gondii ROP1 subverts murine and human innate immune restriction. bioRxiv 2022.03.21.485090. https://doi.org/10.1101/2022.03.21.485090 [Google Scholar] [CrossRef]
Caffaro CE, Boothroyd JC (2011). Evidence for host cells as the major contributor of lipids in the intravacuolar network of toxoplasma-infected cells. Eukaryotic Cell 10: 1095–1099. https://doi.org/10.1128/EC.00002-11 [Google Scholar] [PubMed] [CrossRef]
Caffaro CE, Koshy AA, Liu L, Zeiner GM, Hirschberg CB et al. (2013). A nucleotide sugar transporter involved in glycosylation of the Toxoplasma tissue cyst wall is required for efficient persistence of bradyzoites. PLoS Pathogens 9: e1003331. https://doi.org/10.1371/JOURNAL.PPAT.1003331 [Google Scholar] [PubMed] [CrossRef]
Caldas LA, Attias M, de Souza W (2009). Dynamin inhibitor impairs Toxoplasma gondii invasion. FEMS Microbiology Letters 301: 103–108. [Google Scholar] [PubMed]
Caldas LA, Soares LL, Seabra SH, Attias M, de Souza W (2016). Monitoring of dynamin during the Toxoplasma gondii cell cycle. Pathogens and Disease 74: ftw108. https://doi.org/10.1093/FEMSPD/FTW108 [Google Scholar] [PubMed] [CrossRef]
Camejo A, Gold DA, Lu D, McFetridge K, Julien L et al. (2014). Identification of three novel Toxoplasma gondii rhoptry proteins. International Journal for Parasitology 44: 147–160. https://doi.org/10.1016/J.IJPARA.2013.08.002 [Google Scholar] [PubMed] [CrossRef]
Cardoso R, Nolasco S, Goncąlves J, Cortes HC, Leitaõ A et al. (2014). Besnoitia besnoiti and Toxoplasma gondii: Two apicomplexan strategies to manipulate the host cell centrosome and Golgi apparatus. Parasitology 141: 1436–1454. https://doi.org/10.1017/S0031182014000493 [Google Scholar] [PubMed] [CrossRef]
Cardoso R, Soares H, Hemphill A, Leitão A (2016). Apicomplexans pulling the strings: Manipulation of the host cell cytoskeleton dynamics. Parasitology 143: 957–970. https://doi.org/10.1017/S0031182016000524 [Google Scholar] [PubMed] [CrossRef]
Carey KL, Donahue CG, Ward GE (2000). Identification and molecular characterization of GRA8, a novel, proline-rich, dense granule protein of Toxoplasma gondii. Molecular Biochemical Parasitology 105: 25–37. https://doi.org/10.1016/S0166-6851(99)00160-7 [Google Scholar] [PubMed] [CrossRef]
Carey KL, Jongco AM, Kim K, Ward GE (2004). The Toxoplasma gondii rhoptry protein ROP4 is secreted into the parasitophorous vacuole and becomes phosphorylated in infected cells. Eukaryotic Cell 3: 1320–1330. https://doi.org/10.1128/EC.3.5.1320-1330.2004 [Google Scholar] [PubMed] [CrossRef]
Carruthers V, Boothroyd JC (2007). Pulling together: An integrated model of Toxoplasma cell invasion. Current Opinion in Microbiology 10: 83–89. https://doi.org/10.1016/j.mib.2006.06.017 [Google Scholar] [PubMed] [CrossRef]
Carruthers VB, Giddings OK, Sibley LD (1999). Secretion of micronemal proteins is associated with Toxoplasma invasion of host cells. Cellular Microbiology 1: 225–235. https://doi.org/10.1046/j.1462-5822.1999.00023.x [Google Scholar] [PubMed] [CrossRef]
Carruthers VB, Sibley LD (1997). Sequential protein secretion from three distinct organelles of Toxoplasma gondii accompanies invasion of human fibroblasts. European Journal of Cell Biology 73: 114–123. [Google Scholar] [PubMed]
Carruthers VB, Sibley LD (1999). Mobilization of intracellular calcium stimulates microneme discharge in Toxoplasma gondii. Molecular Microbiology 31: 421–428. https://doi.org/10.1046/J.1365-2958.1999.01174.X [Google Scholar] [PubMed] [CrossRef]
Charron AJ, Sibley LD (2002). Host cells: Mobilizable lipid resources for the intracellular parasite Toxoplasma gondii. Journal of Cell Science 115: 3049–3059. https://doi.org/10.1242/JCS.115.15.3049 [Google Scholar] [PubMed] [CrossRef]
Charron AJ, Sibley LD (2004). Molecular partitioning during host cell penetration by Toxoplasma gondii. Traffic 5: 855–867. https://doi.org/10.1111/j.1600-0854.2004.00228.x [Google Scholar] [PubMed] [CrossRef]
Clough B, Frickel EM (2017). The Toxoplasma parasitophorous vacuole: An evolving host-parasite frontier. Trends in Parasitology 33: 473–488. https://doi.org/10.1016/j.pt.2017.02.007 [Google Scholar] [PubMed] [CrossRef]
Coppens I, Dunn JD, Romano JD, Pypaert M, Zhang H et al. (2006). Toxoplasma gondii sequesters lysosomes from mammalian hosts in the vacuolar space. Cell 125: 261–274. https://doi.org/10.1016/J.CELL.2006.01.056 [Google Scholar] [PubMed] [CrossRef]
Coppens I, Joiner KA (2003). Host but not parasite cholesterol controls Toxoplasma cell entry by modulating organelle discharge. Molecular Biology of the Cell 14: 3804–3820. https://doi.org/10.1091/mbc.E02-12-0830 [Google Scholar] [PubMed] [CrossRef]
Coppens I, Sinai AP, Joiner KA (2000). Toxoplasma gondii exploits host low-density lipoprotein receptor-mediated endocytosis for cholesterol acquisition. The Journal of Cell Biology 149: 167–180. https://doi.org/10.1083/JCB.149.1.167 [Google Scholar] [PubMed] [CrossRef]
Crawford MJ, Thomsen-Zieger N, Ray M, Schachtner J, Roos DS et al. (2006). Toxoplasma gondii scavenges host-derived lipoic acid despite its de novo synthesis in the apicoplast. The EMBO Journal 25: 3214–3222. https://doi.org/10.1038/SJ.EMBOJ.7601189 [Google Scholar] [PubMed] [CrossRef]
Cygan AM, Jean Beltran PM, Mendoza AG, Branon TC, Ting AY et al. (2021). Proximity-labeling reveals novel host and parasite proteins at the Toxoplasma parasitophorous vacuole membrane. mBio 12: e00260–21. https://doi.org/10.1128/MBIO.00260-21 [Google Scholar] [PubMed] [CrossRef]
Cygan AM, Theisen TC, Mendoza AG, Marino ND, Panas MW et al. (2020). Coimmunoprecipitation with MYR1 identifies three additional proteins within the Toxoplasma gondii parasitophorous vacuole required for translocation of dense granule effectors into host cells. mSphere 5: e00858–19. https://doi.org/10.1128/MSPHERE.00858-19 [Google Scholar] [PubMed] [CrossRef]
Dang CV (2012). MYC on the path to cancer. Cell 149: 22–35. https://doi.org/10.1016/J.CELL.2012.03.003 [Google Scholar] [PubMed] [CrossRef]
da Silva CV, da Silva EA, Cruz MC, Chavrier P, Mortara RA (2009). ARF6, PI3-kinase and host cell actin cytoskeleton in Toxoplasma gondii cell invasion. Biochemical Biophysical Research Communications 378: 656–661. https://doi.org/10.1016/j.bbrc.2008.11.108 [Google Scholar] [PubMed] [CrossRef]
de Melo EJT, de Carvalho TU, de Souza W (1992). Penetration of Toxoplasma gondii into host cells induces changes in the distribution of the mitochondria and the endoplasmic reticulum. Cell Structure and Function 17: 311–317. https://doi.org/10.1247/csf.17.311 [Google Scholar] [PubMed] [CrossRef]
de Melo EJT, de Souza W (1996). Pathway of C6-NBD-Ceramide on the host cell infected with Toxoplasma gondii. Cell Structure Function 21: 47–52. https://doi.org/10.1247/CSF.21.47 [Google Scholar] [PubMed] [CrossRef]
de Souza W, Attias M (2015). New views of the Toxoplasma gondii parasitophorous vacuole as revealed by Helium Ion Microscopy (HIM). Journal of Structural Biology 191: 76–85. https://doi.org/10.1016/j.jsb.2015.05.003 [Google Scholar] [PubMed] [CrossRef]
Deffieu MS, Alayi TD, Slomianny C, Tomavo S (2019). The Toxoplasma gondii dense granule protein TgGRA3 interacts with host Golgi and dysregulates anterograde transport. Biology Open 8: e039818. https://doi.org/10.1242/bio.039818 [Google Scholar] [PubMed] [CrossRef]
Denkers EY, Bzik DJ, Fox BA, Butcher BA (2012). An inside job: Hacking into Janus kinase/signal transducer and activator of transcription signaling cascades by the intracellular protozoan Toxoplasma gondii. Infection and Immunity 80: 476–482. https://doi.org/10.1128/IAI.05974-11 [Google Scholar] [PubMed] [CrossRef]
Derouin F, Beauvais B, Lariviere M, Guillot J (1981). Binding of fluorescein-labelled lectins on trophozoites and cysts of 3 strains of Toxoplasma gondii. Comptes Rendus des Seances de la Societe de Biologie et de ses Filiales 175: 761–768. [Google Scholar] [PubMed]
Dongchao Z, Ning J, Qijun C (2020). Loss of rhoptry protein 9 impeded Toxoplasma gondii infectivity. Acta Tropica 207: 105464. https://doi.org/10.1016/J.ACTATROPICA.2020.105464 [Google Scholar] [PubMed] [CrossRef]
Dou Z, McGovern OL, Di Cristina M, Carruthers VB (2014). Toxoplasma gondii ingests and digests host Cytosolic proteins. mBio 5: e01188–14. https://doi.org/10.1128/mBio.01188-14 [Google Scholar] [PubMed] [CrossRef]
Drewry LL, Jones NG, Wang Q, Onken MD, Miller MJ et al. (2019). The secreted kinase ROP17 promotes Toxoplasma gondii dissemination by hijacking monocyte tissue migration. Nature Microbiology 4: 1951–1963. https://doi.org/10.1038/s41564-019-0504-8 [Google Scholar] [PubMed] [CrossRef]
Dubremetz JF, Achbarou A, Bermudes D, Joiner KA (1993). Kinetics and pattern of organelle exocytosis during Toxoplasma gondii/host-cell interaction. Parasitology Research 79: 402–408. https://doi.org/10.1007/BF00931830 [Google Scholar] [PubMed] [CrossRef]
Dunay IR, Gajurel K, Dhakal R, Liesenfeld O, Montoya JG (2018). Treatment of toxoplasmosis: Historical perspective, animal models, and current clinical practice. Clinical Microbiology Review 31. https://doi.org/10.1128/CMR.00057-17 [Google Scholar] [PubMed] [CrossRef]
El Hajj H, Demey E, Poncet J, Lebrun M, Wu B et al. (2006). The ROP2 family of Toxoplasma gondii rhoptry proteins: Proteomic and genomic characterization and molecular modeling. Proteomics 6: 5773–5784. https://doi.org/10.1002/(ISSN)1615-9861 [Google Scholar] [CrossRef]
El Hajj H, Lebrun M, Arold ST, Vial H, Labesse G, et al. (2007a). ROP18 is a rhoptry kinase controlling the intracellular proliferation of Toxoplasma gondii. PLoS Pathogens 3: 0200–0211. https://doi.org/10.1371/journal.ppat.0030014 [Google Scholar] [PubMed] [CrossRef]
El Hajj H, Lebrun M, Fourmaux MN, Vial H, Dubremetz JF (2007b). Inverted topology of the Toxoplasma gondii ROP5 rhoptry protein provides new insights into the association of the ROP2 protein family with the parasitophorous vacuole membrane. Cellular Microbiology 9: 54–64. https://doi.org/10.1111/j.1462-5822.2006.00767.x [Google Scholar] [PubMed] [CrossRef]
Etheridge RD, Alaganan A, Tang K, Lou HJ, Turk BE et al. (2014). The Toxoplasma pseudokinase ROP5 forms complexes with ROP18 and ROP17 kinases that synergize to control acute virulence in mice. Cell Host and Microbe 15: 537–550. https://doi.org/10.1016/j.chom.2014.04.002 [Google Scholar] [PubMed] [CrossRef]
Ferguson DJP (2004). Use of molecular and ultrastructural markers to evaluate stage conversion of Toxoplasma gondii in both the intermediate and definitive host. International Journal for Parasitology 34: 347–360. https://doi.org/10.1016/J.IJPARA.2003.11.024 [Google Scholar] [PubMed] [CrossRef]
Fleckenstein MC, Reese ML, Könen-Waisman S, Boothroyd JC, Howard JC et al. (2012). A toxoplasma gondii pseudokinase inhibits host irg resistance proteins. PLoS Biology 10: 14. https://doi.org/10.1371/journal.pbio.1001358 [Google Scholar] [PubMed] [CrossRef]
Fox BA, Falla A, Rommereim LM, Tomita T, Gigley JP et al. (2011). Type II Toxoplasma gondii KU80 knockout strains enable functional analysis of genes required for cyst development and latent infection. Eukaryotic Cell 10: 1193–1206. https://doi.org/10.1128/EC.00297-10 [Google Scholar] [PubMed] [CrossRef]
Fox BA, Guevara RB, Rommereim LM, Falla A, Bellini V et al. (2019). Toxoplasma gondii parasitophorous vacuole membrane-associated dense granule proteins orchestrate chronic infection and GRA12 underpins resistance to host gamma interferon. mBio 10: e00589–19. https://doi.org/10.1128/mBio.00589-19 [Google Scholar] [PubMed] [CrossRef]
Fox BA, Rommereim LM, Guevara RB, Falla A, Hortua Triana MA et al. (2016). The Toxoplasma gondii rhoptry kinome is essential for chronic infection. mBio 7: e00193–16. https://doi.org/10.1128/MBIO.00193-16 [Google Scholar] [PubMed] [CrossRef]
Franco M, Panas MW, Marino ND, Lee MCW, Buchholz KR et al. (2016). A novel secreted protein, MYR1, is central to Toxoplasma’s manipulation of host cells. mBio 7: e02231–15. https://doi.org/10.1128/mBio.02231-15 [Google Scholar] [PubMed] [CrossRef]
Franco M, Shastri AJ, Boothroyd JC (2014). Infection by Toxoplasma gondii specifically induces host c-Myc and the genes this pivotal transcription factor regulates. Eukaryotic Cell 13: 483–493. https://doi.org/10.1128/EC.00316-13 [Google Scholar] [PubMed] [CrossRef]
Frénal K, Dubremetz JF, Lebrun M, Soldati-Favre D (2017). Gliding motility powers invasion and egress in Apicomplexa. Nature Review Microbiology 15: 645–660. https://doi.org/10.1038/NRMICRO.2017.86 [Google Scholar] [PubMed] [CrossRef]
Frénal K, Soldati-Favre D (2009). Role of the parasite and host cytoskeleton in apicomplexa parasitism. Cell Host and Microbe 5: 602–611. https://doi.org/10.1016/j.chom.2009.05.013 [Google Scholar] [PubMed] [CrossRef]
Fu Y, Brown KM, Jones NG, Moreno SNJ, Sibley LD et al. (2021). Toxoplasma bradyzoites exhibit physiological plasticity of calcium and energy stores controlling motility and egress. eLife 10: 1126. https://doi.org/10.7554/eLife.73011 [Google Scholar] [PubMed] [CrossRef]
Fu Y, Cui X, Fan S, Liu J, Zhang X, et al. (2018). Comprehensive characterization of Toxoplasma acyl coenzyme a-binding protein TgACBP2 and its critical role in parasite cardiolipin metabolism. mBio 9: 1–20. https://doi.org/10.1128/MBIO.01597-18 [Google Scholar] [PubMed] [CrossRef]
Fukumoto J, Sakura T, Matsubara R, Tahara M, Matsuzaki M et al. (2021). Rhoptry kinase protein 39 (ROP39) is a novel factor that recruits host mitochondria to the parasitophorous vacuole of Toxoplasma gondii. Biology Open 10: e058988. https://doi.org/10.1242/BIO.058988 [Google Scholar] [PubMed] [CrossRef]
Garten M, Nasamu AS, Niles JC, Zimmerberg J, Goldberg DE et al. (2018). EXP2 is a nutrient-permeable channel in the vacuolar membrane of Plasmodium and is essential for protein export via PTEX. Nature Microbiology 3: 1090–1098. https://doi.org/10.1038/S41564-018-0222-7 [Google Scholar] [PubMed] [CrossRef]
Gay G, Braun L, Brenier-Pinchart MP, Vollaire J, Josserand V et al. (2016). Toxoplasma gondii TgIST co-opts host chromatin repressors dampening STAT1-dependent gene regulation and IFN-γ-mediated host defenses. Journal of Experimental Medicine 213: 1779–1798. https://doi.org/10.1084/jem.20160340 [Google Scholar] [PubMed] [CrossRef]
Gazzinelli RT, Wysocka M, Hayashi S, Denkers EY, Hieny S et al. (1994). Parasite-induced IL-12 stimulates early IFN-gamma synthesis and resistance during acute infection with Toxoplasma gondii. Journal of Immunol 153: 2533–2543. [Google Scholar]
Gold DA, Kaplan AD, Lis A, Bett GCL, Rosowski EE et al. (2015). The Toxoplasma dense granule proteins GRA17 and GRA23 mediate the movement of small molecules between the host and the parasitophorous vacuole. Cell Host and Microbe 17: 642–652. https://doi.org/10.1016/J.CHOM.2015.04.003 [Google Scholar] [PubMed] [CrossRef]
Goldszmid RS, Coppens I, Lev A, Caspar P, Mellman I et al. (2009). Host ER-parasitophorous vacuole interaction provides a route of entry for antigen cross-presentation in Toxoplasma gondii-infected dendritic cells. Journal of Experimental Medicine 206: 399–410 https://doi.org/10.1084/JEM.20082108 [Google Scholar] [PubMed] [CrossRef]
Gomes AF, Magalhães KG, Rodrigues RM, de Carvalho L, Molinaro R et al. (2014). Toxoplasma gondii-skeletal muscle cells interaction increases lipid droplet biogenesis and positively modulates the production of IL-12, IFN-g and PGE 2. Parasites and Vectors 7: 1–13. https://doi.org/10.1186/1756-3305-7-47 [Google Scholar] [PubMed] [CrossRef]
Guevara RB, Fox BA, Bzik DJ (2020). Toxoplasma gondii parasitophorous vacuole membrane-associated dense granule proteins regulate maturation of the cyst wall. mSphere 5: e00851-19. [Google Scholar] [PubMed]
Guevara RB, Fox BA, Falla A, Bzik DJ (2019). Toxoplasma gondii intravacuolar-network-associated dense granule proteins regulate maturation of the cyst matrix and cyst wall. mSphere 4: e00487–19. https://doi.org/10.1128/MSPHERE.00487-19 [Google Scholar] [PubMed] [CrossRef]
Guimarães EV, Acquarone M, de Carvalho L, Barbosa HS (2007). Anionic sites on Toxoplasma gondii tissue cyst wall: Expression, uptake and characterization. Micron 38: 651–658. https://doi.org/10.1016/J.MICRON.2006.09.002 [Google Scholar] [PubMed] [CrossRef]
Guérin A, Corrales RM, Parker ML, Lamarque MH, Jacot D et al. (2017). Efficient invasion by Toxoplasma depends on the subversion of host protein networks. Nature Microbiology 2: 1358–1366. https://doi.org/10.1038/S41564-017-0018-1 [Google Scholar] [PubMed] [CrossRef]
Hakimi MA, Olias P, Sibley LD (2017). Toxoplasma effectors targeting host signaling and transcription. Clinical Microbiology Review 30: 615–644. https://doi.org/10.1128/CMR.00005-17 [Google Scholar] [PubMed] [CrossRef]
Halonen SK, Weidner E (1994). Overcoating of Toxoplasma parasitophorous vacuoles with host cell vimentin type intermediate filaments. The of Journal Eukaryot Microbiology 41: 65–71. https://doi.org/10.1111/J.1550-7408.1994.TB05936.X [Google Scholar] [PubMed] [CrossRef]
Halonen S, Weiss L (2013). Toxoplasmosis. Handbook of Clinical Neurology 114: 125–145. https://doi.org/10.1016/B978-0-444-53490-3.00008-X [Google Scholar] [PubMed] [CrossRef]
He H, Brenier-Pinchart MP, Braun L, Kraut A, Touquet B et al. (2018). Characterization of a Toxoplasma effector uncovers an alternative GSK3/β-catenin-regulatory pathway of inflammation. eLife 7: e39887. https://doi.org/10.7554/ELIFE.39887 [Google Scholar] [PubMed] [CrossRef]
He C, Kong L, Zhou L, Xia J, Wei H et al. (2017). Host cell vimentin restrains Toxoplasma gondii invasion and phosphorylation of vimentin is partially regulated by interaction with Tg ROP18. International Journal of Biological Sciences 13: 1126–1137. https://doi.org/10.7150/IJBS.21247 [Google Scholar] [PubMed] [CrossRef]
Hermanns T, Müller UB, Könen-Waisman S, Howard JC, Steinfeldt T (2016). The Toxoplasma gondii rhoptry protein ROP18 is an Irga6-specific kinase and regulated by the dense granule protein GRA7. Cellular Microbiology 18: 244–259. https://doi.org/10.1111/CMI.12499 [Google Scholar] [PubMed] [CrossRef]
Horta MF, Andrade LO, Martins-Duarte É.S, Castro-Gomes T (2020). Cell invasion by intracellular parasites-the many roads to infection. Journal of Cell Science 133: jcs232488. https://doi.org/10.1242/jcs.232488 [Google Scholar] [PubMed] [CrossRef]
Huynh MH, Carruthers VB (2006). Toxoplasma MIC2 is a major determinant of invasion and virulence. PLoS Pathogens 2: 753–762. https://doi.org/10.1371/JOURNAL.PPAT.0020084 [Google Scholar] [PubMed] [CrossRef]
Ihara F, Fereig RM, Himori Y, Kameyama K, Umeda K et al. (2020). Toxoplasma gondii dense granule proteins 7, 14, and 15 are involved in modification and control of the immune response mediated via NF-κB pathway. Frontiers in Immunology 11: 1709. https://doi.org/10.3389/FIMMU.2020.01709 [Google Scholar] [PubMed] [CrossRef]
Ivashkiv LB (2018). IFNγ: signalling, epigenetics and roles in immunity, metabolism, disease and cancer immunotherapy. Nature Reviews Immunology 18: 545–558. DOI10.1038/S41577-018-0029-Z. [Google Scholar] [PubMed] [CrossRef]
Jones TC, Hirsch JG (1972). The interaction between Toxoplasma gondii and mammalian cells. II. The absence of lysosomal fusion with phagocytic vacuoles containing living parasites. The Journal of Experimental Medicine 136: 1173–1194. https://doi.org/10.1084/JEM.136.5.1173 [Google Scholar] [PubMed] [CrossRef]
Katris NJ, Ke H, McFadden GI, van Dooren GG, Waller RF (2019). Calcium negatively regulates secretion from dense granules in Toxoplasma gondii. Cellular Microbiology 21: 13011. https://doi.org/10.1111/CMI.13011 [Google Scholar] [PubMed] [CrossRef]
Kelly FD, Wei BM, Cygan AM, Parker ML, Boulanger MJ et al. (2017). Toxoplasma gondii MAF1b binds the host cell MIB complex to mediate mitochondrial association. mSphere 2: e00183–17. https://doi.org/10.1128/MSPHERE.00183-17 [Google Scholar] [PubMed] [CrossRef]
Kim JY, Ahn HJ, Ryu KJ, Nam HW (2008). Interaction between parasitophorous vacuolar membrane-associated GRA3 and calcium modulating ligand of host cell endoplasmic reticulum in the parasitism of Toxoplasma gondii. The Korean Journal of Parasitology 46: 209–216. https://doi.org/10.3347/KJP.2008.46.4.209 [Google Scholar] [PubMed] [CrossRef]
Kim SK, Boothroyd JC (2005). Stage-specific expression of surface antigens by Toxoplasma gondii as a mechanism to facilitate parasite persistence. Journal of Immunology 174: 8038–8048. https://doi.org/10.4049/JIMMUNOL.174.12.8038 [Google Scholar] [PubMed] [CrossRef]
Kim SK, Fouts AE, Boothroyd JC (2007). Toxoplasma gondii dysregulates IFN-gamma-inducible gene expression in human fibroblasts: Insights from a genome-wide transcriptional profiling. Journal of Immunology 178: 5154–5165. https://doi.org/10.4049/JIMMUNOL.178.8.5154 [Google Scholar] [PubMed] [CrossRef]
Kim EW, Nadipuram SM, Tetlow AL, Barshop WD, Liu PT et al. (2016). The rhoptry pseudokinase ROP54 modulates Toxoplasma gondii virulence and host GBP2 loading. mSphere 1: e00045–16. https://doi.org/10.1128/MSPHERE.00045-16 [Google Scholar] [PubMed] [CrossRef]
Kochanowsky JA, Thomas KK, Koshy AA (2021). Rop16-mediated activation of stat6 suppresses host cell reactive oxygen species production, facilitating type III Toxoplasma gondii growth and survival. mBio 12: 1–17. https://doi.org/10.1128/mBio.03305-20 [Google Scholar] [PubMed] [CrossRef]
Koning-Ward TF, de Gilson PR, Boddey JA, Rug M, Smith BJ et al. (2009). A newly discovered protein export machine in malaria parasites. Nature 459: 945–949. https://doi.org/10.1038/nature08104 [Google Scholar] [PubMed] [CrossRef]
Labesse G, Gelin M, Bessin Y, Lebrun M, Papoin J et al. (2009). ROP2 from Toxoplasma gondii: A virulence factor with a protein-kinase fold and no enzymatic activity. Structure 17: 139–146. https://doi.org/10.1016/J.STR.2008.11.005 [Google Scholar] [PubMed] [CrossRef]
Labruyere E, Lingnau M, Mercier C, Sibley LD (1999). Differential membrane targeting of the secretory proteins GRA4 and GRA6 within the parasitophorous vacuole formed by Toxoplasma gondii. Molecular Biochemical Parasitology 102: 311–324. https://doi.org/10.1016/S0166-6851(99)00092-4 [Google Scholar] [PubMed] [CrossRef]
LaFavers KA, Márquez-Nogueras KM, Coppens I, Moreno SNJ, Arrizabalaga G (2017). A novel dense granule protein, GRA41, regulates timing of egress and calcium sensitivity in Toxoplasma gondii. Cellular Microbiology 19: 12749. https://doi.org/10.1111/CMI.12749 [Google Scholar] [PubMed] [CrossRef]
Lecordier L, Mercier C, David Sibley L, Cesbron-Delauwz MF (1999). Transmembrane insertion of the Toxoplasma gondii GRA5 protein occurs after soluble secretion into the host cell. Molecular Biology of the Cell 10: 1277–1287. https://doi.org/10.1091/MBC.10.4.1277 [Google Scholar] [PubMed] [CrossRef]
Lecordier L, Moleon-Borodowsky I, Dubremetz JF, Tourvieille B, Mercier C et al. (1995). Characterization of a dense granule antigen of Toxoplasma gondii (GRA6) associated to the network of the parasitophorous vacuole. Molecular Biochemical Parasitology 70: 85–94. https://doi.org/10.1016/0166-6851(95)00010-X [Google Scholar] [PubMed] [CrossRef]
Lemgruber L, de Souza W, Vommaro RC (2008). Freeze-fracture study of the dynamics of Toxoplasma gondii parasitophorous vacuole development. Micron 39: 177–183. https://doi.org/10.1016/j.micron.2007.01.002 [Google Scholar] [PubMed] [CrossRef]
Lemgruber L, Lupetti P, Martins-Duarte ES, de Souza W, Vommaro RC (2011). The organization of the wall filaments and characterization of the matrix structures of Toxoplasma gondii cyst form. Cellular Microbiology 13: 1920–1932. https://doi.org/10.1111/J.1462-5822.2011.01681.X [Google Scholar] [PubMed] [CrossRef]
Lentini G, El Hajj H, Papoin J, Fall G, Pfaff AW et al. (2017). Characterization of Toxoplasma DegP, a rhoptry serine protease crucial for lethal infection in mice. PLoS One 12: e0189556. https://doi.org/10.1371/JOURNAL.PONE.0189556 [Google Scholar] [PubMed] [CrossRef]
Lige B, Romano JD, Bandaru VV, Ehrenman K, Levitskaya J, Sampels V, Haughey NJ, Coppens I (2011). Deficiency of a Niemann-Pick, type C1-related protein in toxoplasma is associated with multiple lipidoses and increased pathogenicity. PLoS Pathogens 7: e1002410. https://doi.org/10.1371/journal.ppat.1002410 [Google Scholar] [PubMed] [CrossRef]
Lopez J, Bittame A, Massera C, Vasseur V, Effantin G et al. (2015). Intravacuolar membranes regulate CD8 T cell recognition of membrane-bound Toxoplasma gondii protective antigen. Cell Reports 13: 2273–2286. https://doi.org/10.1016/j.celrep.2015.11.001 [Google Scholar] [PubMed] [CrossRef]
Lourido S, Moreno SNJ (2015). The calcium signaling toolkit of the Apicomplexan parasites Toxoplasma gondii and Plasmodium spp. Cell Calcium 57: 186–193. https://doi.org/10.1016/J.CECA.2014.12.010 [Google Scholar] [PubMed] [CrossRef]
Ma JS, Sasai M, Ohshima J, Lee Y, Bando H et al. (2014). Selective and strain-specific NFAT4 activation by the Toxoplasma gondii polymorphic dense granule protein GRA6. The Journal of Experimental Medicine 211: 2013–2032. https://doi.org/10.1084/JEM.20131272 [Google Scholar] [PubMed] [CrossRef]
Magno RC, Lemgruber L, Vommaro RC, de Souza W, Attias M (2005). Intravacuolar network may act as a mechanical support for Toxoplasma gondii inside the parasitophorous vacuole. Microscopy Research Technique 67: 45–52. https://doi.org/10.1002/jemt.20182 [Google Scholar] [PubMed] [CrossRef]
Marino ND, Panas MW, Franco M, Theisen TC, Naor A et al. (2018). Identification of a novel protein complex essential for effector translocation across the parasitophorous vacuole membrane of Toxoplasma gondii. PLoS Pathogens 14: e1006828. https://doi.org/10.1371/JOURNAL.PPAT.1006828 [Google Scholar] [PubMed] [CrossRef]
Martinez FO, Gordon S (2014). The M1 and M2 paradigm of macrophage activation: Time for reassessment. F1000prime Reports 6: 13. https://doi.org/10.12703/P6-13 [Google Scholar] [PubMed] [CrossRef]
Matta SK, Olias P, Huang Z, Wang Q, Park E et al. (2019). Toxoplasma gondii effector TgIST blocks type I interferon signaling to promote infection. Proceedings of the National Academy of Sciences of the United States of America 116: 17480–17491. https://doi.org/10.1073/pnas.1904637116 [Google Scholar] [PubMed] [CrossRef]
Mayoral J, Guevara RB, Rivera-Cuevas Y, Tu V, Tomita T et al. (2022). Dense granule protein GRA64 interacts with host cell ESCRT proteins during Toxoplasma gondii infection. mBio 4: e01442–22. https://doi.org/10.1128/MBIO.01442-22 [Google Scholar] [PubMed] [CrossRef]
Mayoral J, Shamamian P, Weiss LM (2020). In vitro characterization of protein effector export in the bradyzoite stage of Toxoplasma gondii. mBio 11: 00046–20. https://doi.org/10.1128/MBIO.00046-20 [Google Scholar] [PubMed] [CrossRef]
Melo EJT, Carvalho TMU, De Souza W (2001). Behaviour of microtubules in cells infected with Toxoplasma gondii. BIOCELL 25: 53–59. [Google Scholar] [PubMed]
Melo E, de Souza W (1996). Pathway of C6-NBD-ceramide on the host cell infected with Toxoplasma gondii. Cell Structure and Function 21: 47–52. https://doi.org/10.1247/csf.21.47 [Google Scholar] [PubMed] [CrossRef]
Melo EJT, De Souza W (1997). Relationship between the host cell endoplasmic reticulum and the parasitophorous vacuole containing Toxoplasma gondii. Cell Structure and Function 22: 317–323. https://doi.org/10.1247/CSF.22.317 [Google Scholar] [PubMed] [CrossRef]
Mercer HL, Snyder LM, Doherty CM, Fox BA, Bzik DJ et al. (2020). Toxoplasma gondii dense granule protein GRA24 drives MyD88-independent p38 MAPK activation, IL-12 production and induction of protective immunity. PLoS Pathogens 16: e1008572. https://doi.org/10.1371/JOURNAL.PPAT.1008572 [Google Scholar] [PubMed] [CrossRef]
Mercier C, Adjogble KDZ, Däubener W, Delauw MFC (2005). Dense granules: Are they key organelles to help understand the parasitophorous vacuole of all apicomplexa parasites? International Journal for Parasitology 35: 829–849. https://doi.org/10.1016/j.ijpara.2005.03.011 [Google Scholar] [PubMed] [CrossRef]
Mercier C, Cesbron-Delauw MF (2015). Toxoplasma secretory granules: One population or more? Trends in Parasitology 31: 60–71. https://doi.org/10.1016/j.pt.2014.12.002 [Google Scholar] [PubMed] [CrossRef]
Mercier C, Dubremetz JF, Rauscher B, Lecordier L, Sibley LD et al. (2002). Biogenesis of nanotubular network in Toxoplasma parasitophorous vacuole induced by parasite proteins. Molecular Biology of the Cell 13: 2397–2409. https://doi.org/10.1091/mbc.E02-01-0021 [Google Scholar] [PubMed] [CrossRef]
Michelin A, Bittame A, Bordat Y, Travier L, Mercier C et al. (2009). GRA12, a Toxoplasma dense granule protein associated with the intravacuolar membranous nanotubular network. International Journal for Parasitology 39: 299–306. https://doi.org/10.1016/j.ijpara.2008.07.011 [Google Scholar] [PubMed] [CrossRef]
Milligan-Myhre K, Wilson SK, Knoll LJ (2016). Developmental change in translation initiation alters the localization of a common microbial protein necessary for Toxoplasma chronic infection. Molecular Microbiology 102: 1086–1098. https://doi.org/10.1111/MMI.13538 [Google Scholar] [PubMed] [CrossRef]
Molestina RE, El-guendy N, Sinai AP (2008). Infection with Toxoplasma gondii results in dysregulation of the host cell cycle. Cellular Microbiology 10: 1153–1165. https://doi.org/10.1111/J.1462-5822.2008.01117.X [Google Scholar] [PubMed] [CrossRef]
Montoya J, Liesenfeld O (2004). Toxoplasmosis. Lancet 363: 1965–1976. https://doi.org/10.1016/S0140-6736(04)16412-X [Google Scholar] [PubMed] [CrossRef]
Mordue DG, Desai N, Dustin M, Sibley LD (1999a). Invasion by Toxoplasma gondii establishes a moving junction that selectively excludes host cell plasma membrane proteins on the basis of their membrane anchoring. The Journal of Experimental Medicine 190: 1783–1792. https://doi.org/10.1084/jem.190.12.1783 [Google Scholar] [PubMed] [CrossRef]
Mordue DG, Håkansson S, Niesman I, David Sibley L (1999b). Toxoplasma gondii resides in a vacuole that avoids fusion with host cell endocytic and exocytic vesicular trafficking pathways. Experimental Parasitology 92: 87–99. https://doi.org/10.1006/expr.1999.4412 [Google Scholar] [PubMed] [CrossRef]
Mordue DG, Sibley LD (1997). Intracellular fate of vacuoles containing Toxoplasma gondii is determined at the time of formation and depends on the mechanism of entry. Journal of Immunology 159. https://doi.org/10.4049/jimmunol.159.9.4452 [Google Scholar] [CrossRef]
Mota LAM, Roberto-Neto J, Monteiro VG, Lobato CSS, de Oliveira MA et al. (2014). Culture of mouse peritoneal macrophages with mouse serum induces lipid bodies that associate with the parasitophorous vacuole and decrease their microbicidal capacity against Toxoplasma gondii. Memórias do Instituto Oswaldo Cruz 109: 767–774. https://doi.org/10.1590/0074-0276140119 [Google Scholar] [PubMed] [CrossRef]
Na RH, Zhu GH, Luo JX, Meng XJ, Cui L et al. (2013). Enzymatically active Rho and Rac small-GTPases are involved in the establishment of the vacuolar membrane after Toxoplasma gondii invasion of host cells. BMC Microbiology 13: 125. https://doi.org/10.1186/1471-2180-13-125 [Google Scholar] [PubMed] [CrossRef]
Nadipuram SM, Kim EW, Vashisht AA, Lin AH, Bell HN et al. (2016). In vivo biotinylation of the Toxoplasma parasitophorous vacuole reveals novel dense granule proteins important for parasite growth and pathogenesis. mBio 7: e00808–16. https://doi.org/10.1128/MBIO.00808-16 [Google Scholar] [PubMed] [CrossRef]
Nadipuram SM, Thind AC, Rayatpisheh S, Wohlschlegel JA, Bradley PJ (2020). Proximity biotinylation reveals novel secreted dense granule proteins of Toxoplasma gondii bradyzoites. PLoS One 15: e0232552. https://doi.org/10.1371/JOURNAL.PONE.0232552 [Google Scholar] [PubMed] [CrossRef]
Naor A, Panas MW, Marino N, Coffey MJ, Tonkin CJ et al. (2018). MYR1-dependent effectors are the major drivers of a host cell’s early response to Toxoplasma, including counteracting MYR1-independent effects. mBio 9: e02401–17. https://doi.org/10.1128/MBIO.02401-17 [Google Scholar] [PubMed] [CrossRef]
Nolan SJ, Romano JD, Coppens I (2017). Host lipid droplets: An important source of lipids salvaged by the intracellular parasite Toxoplasma gondii. PLoS Pathogens 13: e1006362. https://doi.org/10.1371/JOURNAL.PPAT.1006362 [Google Scholar] [PubMed] [CrossRef]
Nyonda MA, Hammoudi PM, Ye S, Maire J, Marq JB et al. (2021). Toxoplasma gondii GRA60 is an effector protein that modulates host cell autonomous immunity and contributes to virulence. Cellular Microbiology 23: e13278. https://doi.org/10.1111/CMI.13278 [Google Scholar] [PubMed] [CrossRef]
Okada T, Marmansari D, Li ZM, Adilbish A, Canko S et al. (2013). A novel dense granule protein, GRA22, is involved in regulating parasite egress in Toxoplasma gondii. Molecular Biochemical Parasitology 189: 5–13. https://doi.org/10.1016/J.MOLBIOPARA.2013.04.005 [Google Scholar] [PubMed] [CrossRef]
Olias P, Etheridge RD, Zhang Y, Holtzman MJ, Sibley LD (2016). Toxoplasma effector recruits the Mi-2/NuRD complex to repress STAT1 transcription and block IFN-γ-dependent gene expression. Cell Host and Microbe 20: 72–82. https://doi.org/10.1016/J.CHOM.2016.06.006 [Google Scholar] [PubMed] [CrossRef]
Olmos Y (2022). The ESCRT machinery: Remodeling, repairing, and sealing membranes. Membranes 12: 633. https://doi.org/10.3390/MEMBRANES12060633 [Google Scholar] [PubMed] [CrossRef]
Ong YC, Reese ML, Boothroyd JC (2010). Toxoplasma rhoptry protein 16 (ROP16) subverts host function by direct tyrosine phosphorylation of STAT6. Journal of Biological Chemistry 285: 28731–28740. https://doi.org/10.1074/jbc.M110.112359 [Google Scholar] [PubMed] [CrossRef]
Panas MW, Boothroyd JC (2020). Toxoplasma uses GRA16 To upregulate host c-Myc. mSphere 5: e00402–20. https://doi.org/10.1128/msphere.00402-20 [Google Scholar] [PubMed] [CrossRef]
Panas MW, Ferrel A, Naor A, Tenborg E, Lorenzi HA (2019a). Translocation of dense granule effectors across the parasitophorous vacuole membrane in Toxoplasma-Infected cells requires the activity of ROP17, a rhoptry protein kinase. mSphere, e00276–19. https://doi.org/10.1128/MSPHERE.00276-19 [Google Scholar] [PubMed] [CrossRef]
Panas MW, Naor A, Cygan AM, Boothroyd JC (2019b). Toxoplasma controls host cyclin E expression through the use of a novel myr1-dependent effector protein HCE1. mBio 10: e00674–19. https://doi.org/10.1128/mBio.00674-19 [Google Scholar] [PubMed] [CrossRef]
Pappas G, Roussos N, Falagas ME (2009). Toxoplasmosis snapshots: Global status of Toxoplasma gondii seroprevalence and implications for pregnancy and congenital toxoplasmosis. Internatinal Journal for Parasitology 39: 1385–1394. https://doi.org/10.1016/j.ijpara.2009.04.003 [Google Scholar] [PubMed] [CrossRef]
Paredes-Santos TC, Martins-Duarte ES, de Souza W, Attias M, Vommaro RC (2018). Toxoplasma gondii reorganizes the host cell architecture during spontaneous cyst formation in vitro. Parasitology 145: 1027–1038. [Google Scholar] [PubMed]
Paredes-Santos T, Wang Y, Waldman B, Lourido S, Saeij JP (2019). The GRA17 parasitophorous vacuole membrane permeability pore contributes to bradyzoite viability. Frontiers in Cellular and Infection Microbiology 9: 321. https://doi.org/10.3389/fcimb.2019.00321 [Google Scholar] [PubMed] [CrossRef]
Parmley SF, Yang S, Harth G, David Sibley L, Sucharczuk A et al. (1994). Molecular characterization of a 65-kilodalton Toxoplasma gondii antigen expressed abundantly in the matrix of tissue cysts. Molecular Biochemical Parasitology 66: 283–296. https://doi.org/10.1017/S0031182017002050 [Google Scholar] [PubMed] [CrossRef]
Pavlou G, Biesaga M, Touquet B, Lagal V, Balland M et al. (2018). Toxoplasma parasite twisting motion mechanically induces host cell membrane fission to complete invasion within a protective vacuole. Cell Host and Microbe 24: 81–96.e5. https://doi.org/10.1016/0166-6851(94)90155-4 [Google Scholar] [PubMed] [CrossRef]
Peixoto L, Chen F, Harb OS, Davis PH, Beiting DP et al. (2010). Integrative genomic approaches highlight a family of parasite-specific kinases that regulate host responses. Cell Host and Microbe 8: 208–218. https://doi.org/10.1016/J.CHOM.2018.06.003 [Google Scholar] [CrossRef]
Pernas L, Adomako-Ankomah Y, Shastri AJ, Ewald SE, Treeck M et al. (2014). Toxoplasma effector MAF1 mediates recruitment of host mitochondria and impacts the host response. PLoS Biology 12: e1001845. https://doi.org/10.1371/JOURNAL.PBIO.1001845 [Google Scholar] [PubMed] [CrossRef]
Porchet-Hennere E, Torpier G (1983). Relations entre Toxoplasma et sa cellule-hote. Protistologica. https://www.researchgate.net/publication/284757407_Relations_entre_Toxoplasma_et_sa_cellule-hote. [Google Scholar]
Rastogi S, Cygan AM, Boothroyd JC (2019). Translocation of effector proteins into host cells by Toxoplasma gondii. Current Opinion in Microbiology 52: 130–138. https://doi.org/10.1016/J.MIB.2019.07.002 [Google Scholar] [PubMed] [CrossRef]
Reese ML, Shah, Boothroyd JC (2014). The Toxoplasma pseudokinase ROP5 is an allosteric inhibitor of the immunity-related GTPases. The Journal of Biological Chemistry 289: 27849–27858. https://doi.org/10.1074/JBC.M114.567057 [Google Scholar] [PubMed] [CrossRef]
Reichmann G, Długońska H, Fischer HG (2002). Characterization of TgROP9 (p36a novel rhoptry protein of Toxoplasma gondii tachyzoites identified by T cell clone. Molecular Biochemical Parasitology 119: 43–54. https://doi.org/10.1016/S0166-6851(01)00397-8 [Google Scholar] [PubMed] [CrossRef]
Rivera-Cuevas Y, Mayoral J, di Cristina M et al. (2021). Toxoplasma gondii exploits the host ESCRT machinery for parasite uptake of host cytosolic proteins. PLoS Pathogens 17: e1010138. https://doi.org/10.1371/journal.ppat.1010138 [Google Scholar] [PubMed] [CrossRef]
Romano JD, Coppens I (2013). Host organelle hijackers: A similar modus operandi for Toxoplasma gondii and chlamydia trachomatis: Co-infection model as a tool to investigate pathogenesis. Pathogens and Disease 69: 72–86. https://doi.org/10.1111/2049-632X.12057 [Google Scholar] [PubMed] [CrossRef]
Romano JD, Nolan SJ, Porter C, Ehrenman K, Hartman EJ et al. (2017). The parasite Toxoplasma sequesters diverse Rab host vesicles within an intravacuolar network. Journal of Cell Biology 216: 4235–4254. https://doi.org/10.1083/jcb.201701108 [Google Scholar] [PubMed] [CrossRef]
Romano JD, Sonda S, Bergbower E, Smith ME, Coppens I (2013). Toxoplasma gondii salvages sphingolipids from the host Golgi through the rerouting of selected Rab vesicles to the parasitophorous vacuole. Molecular Biology of the Cell 24: 1974–1995. https://doi.org/10.1091/mbc.e12-11-0827 [Google Scholar] [PubMed] [CrossRef]
Rome ME, Beck JR, Turetzky JM, Webster P, Bradley PJ (2008). Intervacuolar transport and unique topology of GRA14, a novel dense granule protein in Toxoplasma gondii. Infection and Immunity 76: 4865–4875. https://doi.org/10.1128/IAI.00782-08 [Google Scholar] [PubMed] [CrossRef]
Rommereim LM, Bellini V, Fox BA, Pètre G, Rak C et al. (2016). Phenotypes associated with knockouts of eight dense granule gene loci (GRA2-9) in virulent Toxoplasma gondii. PLoS One 11: e0159306. https://doi.org/10.1371/journal.pone.0159306 [Google Scholar] [PubMed] [CrossRef]
Rommereim LM, Fox BA, Butler KL, Cantillana V, Taylor GA et al. (2019). Rhoptry and dense granule secreted effectors regulate CD8 + T cell recognition of Toxoplasma gondii infected host cells. Frontiers in Immunology 10: 2104. https://doi.org/10.3389/fimmu.2019.02104 [Google Scholar] [PubMed] [CrossRef]
Rosenberg A, Sibley LD (2021). Toxoplasma gondii secreted effectors co-opt host repressor complexes to inhibit necroptosis. Cell Host and Microbe 29: 1186–1198.e8. https://doi.org/10.1016/j.chom.2021.04.016 [Google Scholar] [PubMed] [CrossRef]
Rosowski EE, Lu D, Julien L, Rodda L, Gaiser RA et al. (2011). Strain-specific activation of the NF-κB pathway by GRA15, a novel Toxoplasma gondii dense granule protein. The Journal of Experimental Medicine 208: 195–212. https://doi.org/10.1084/jem.20100717 [Google Scholar] [PubMed] [CrossRef]
Rudzki EN, Ander SE, Coombs RS, Alrubaye HS, Cabo LF et al. (2021). Toxoplasma gondii GRA28 is required for placenta-specific induction of the regulatory chemokine CCL22 in human and mouse. mBio 12: e01591–21. https://doi.org/10.1128/MBIO.01591-21 [Google Scholar] [PubMed] [CrossRef]
Rêgo WMF, Costa JGL, Baraviera RCA, Pinto LV, Bessa GL et al. (2017). Association of ROP18 and ROP5 was efficient as a marker of virulence in atypical isolates of Toxoplasma gondii obtained from pigs and goats in Piauí. Brazil Veterinary Parasitology 247: 19–25. https://doi.org/10.1016/j.vetpar.2017.09.015 [Google Scholar] [PubMed] [CrossRef]
Saeij JPJ, Boyle JP, Coller S, Taylor S, Sibley LD et al. (2006). Polymorphic secreted kinases are key virulence factors in toxoplasmosis. Science 314: 1780–1783. https://doi.org/10.1126/SCIENCE.1133690 [Google Scholar] [PubMed] [CrossRef]
Saeij JPJ, Coller S, Boyle JP, Jerome ME, White MW et al. (2007). Toxoplasma co-opts host gene expression by injection of a polymorphic kinase homologue. Nature 445: 324–327. https://doi.org/10.1038/NATURE05395 [Google Scholar] [PubMed] [CrossRef]
Sanchez SG, Besteiro S (2021). The pathogenicity and virulence of Toxoplasma gondii. Virulence 12: 3095–3114. https://doi.org/10.1080/21505594.2021.2012346 [Google Scholar] [PubMed] [CrossRef]
Sangaré LO, Ólafsson EB, Wang Y, Yang N, Julien L et al. (2019). In Vivo CRISPR screen identifies TgWIP as a Toxoplasma modulator of dendritic cell migration. Cell Host and Microbe 26: 478–492.e8. [Google Scholar]
Sasai M, Yamamoto M (2019). Innate, adaptive, and cell-autonomous immunity against Toxoplasma gondii infection. Experimental & Molecular Medicine 51: 1–10, 2019. https://doi.org/10.1038/s12276-019-0353-9 [Google Scholar] [PubMed] [CrossRef]
Schwab JC, Beckers CJM, Joiner KA (1994). The parasitophorous vacuole membrane surrounding intracellular Toxoplasma gondii functions as a molecular sieve. Proceedings of the National Academy of Sciences of the United States of America 91: 509–513. https://doi.org/10.1073/pnas.91.2.509 [Google Scholar] [PubMed] [CrossRef]
Shastri AJ, Marino ND, Franco M, Lodoen MB, Boothroyd JC (2014). GRA25 is a novel virulence factor of Toxoplasma gondii and influences the host immune response. Infection and Immunity 82: 2595–2605. https://doi.org/10.1128/IAI.01339-13 [Google Scholar] [PubMed] [CrossRef]
Sher A, Collazzo C, Scanga C, Jankovic D, Yap G et al. (2003). Induction and regulation of IL-12-dependent host resistance to Toxoplasma gondii. Immunologic Research 27: 521–527. https://doi.org/10.1385/IR:27:2-3:521 [Google Scholar] [PubMed] [CrossRef]
Shwab EK, Jiang T, Pena HFJ, Gennari SM, Dubey JP et al. (2016). The ROP18 and ROP5 gene allele types are highly predictive of virulence in mice across globally distributed strains of Toxoplasma gondii. International Journal for Parasitology 46: 141–146. https://doi.org/10.1016/J.IJPARA.2015.10.005 [Google Scholar] [PubMed] [CrossRef]
Sibley LD, Håkansson S, Carruthers VB (1998). Gliding motility: An efficient mechanism for cell penetration. Current Biology 8: R12–R14. https://doi.org/10.1016/S0960-9822(98)70008-9 [Google Scholar] [PubMed] [CrossRef]
Sibley LD, Niesman IR, Parmley SF, Cesbron-Delauw MF (1995). Regulated secretion of multi-lamellar vesicles leads to formation of a tubulo-vesicular network in host-cell vacuoles occupied by Toxoplasma gondii. Journal of Cell Science 108: 1669–1677. https://doi.org/10.1242/JCS.108.4.1669 [Google Scholar] [PubMed] [CrossRef]
Sibley LD, Weidner E, Krahenbuhl JL (1985). Phagosome acidification blocked by intracellular Toxoplasma gondii. Nature 315: 416–419. https://doi.org/10.1038/315416A0 [Google Scholar] [PubMed] [CrossRef]
Sinai AP (2013). The Toxoplasma gondii parasitophorous vacuole membrane. A multifunctional organelle in the infected cell. Toxoplasma Gondii (Second EditionThe Model Apicomplexan-Perspectives and Methods 375–387. https://doi.org/10.1016/B978-0-12-396481-6.00011-8 [Google Scholar] [CrossRef]
Sinai AP, Webster P, Joiner KA (1997). Association of host cell endoplasmic reticulum and mitochondria with the Toxoplasma grondii parasitophorous vacuole membrane: A high affinity interaction. Journal of Cell Science 110: 2117–2128. https://doi.org/10.1242/jcs.110.17.2117 [Google Scholar] [PubMed] [CrossRef]
Sit ST, Manser E (2011). Rho GTPases and their role in organizing the actin cytoskeleton. Journal of Cell Science 124: 679–683. https://doi.org/10.1242/JCS.064964 [Google Scholar] [PubMed] [CrossRef]
Soldati D, Dubremetz JF, Lebrun M (2001). Microneme proteins: Structural and functional requirements to promote adhesion and invasion by the apicomplexan parasite Toxoplasma gondii. International Journal for Parasitology 31: 1293–1302. https://doi.org/10.1016/S0020-7519(01)00257-0 [Google Scholar] [PubMed] [CrossRef]
Steinfeldt T, Könen-Waisman S, Tong L, Pawlowski N, Lamkemeyer T et al. (2010). Phosphorylation of mouse immunity-related gtpase (IRG) resistance proteins is an evasion strategy for virulent Toxoplasma gondii. PLoS Biology 8: e1000576. https://doi.org/10.1371/JOURNAL.PBIO.1000576 [Google Scholar] [PubMed] [CrossRef]
Suss-Toby E, Zimmerberg J, Ward GE (1996). Toxoplasma invasion: The parasitophorous vacuole is formed from host cell plasma membrane and pinches off via a fission pore. Proceedings of the National Academy of Sciences of the United States of America 93: 8413–8418. https://doi.org/10.1073/pnas.93.16.8413 [Google Scholar] [PubMed] [CrossRef]
Sweeney KR, Morrissette NS, Lachapelle S, Blader IJ (2010). Host cell invasion by Toxoplasma gondii is temporally regulated by the host microtubule cytoskeleton. Eukaryotic Cell 9: 1680–1689. https://doi.org/10.1128/EC.00079-10 [Google Scholar] [PubMed] [CrossRef]
Syn G, Anderson D, Blackwell JM, Jamieson SE (2017). Toxoplasma gondii infection is associated with mitochondrial dysfunction in-vitro. Frontiers in Cellular and Infection Microbiology 7: 512. https://doi.org/10.3389/FCIMB.2017.00512 [Google Scholar] [PubMed] [CrossRef]
Taylor GA (2007). IRG proteins: Key mediators of interferon-regulated host resistance to intracellular pathogens. Cellular Microbiology 9. https://doi.org/10.1111/j.1462-5822.2007.00916.x [Google Scholar] [PubMed] [CrossRef]
ten Hoeve AL, Braun L, Rodriguez ME, Olivera GC, Bougdour A et al. (2022). The Toxoplasma effector GRA28 promotes parasite dissemination by inducing dendritic cell-like migratory properties in infected macrophages. Cell Host and Microbe 1-19. https://doi.org/10.1016/J.CHOM.2022.10.001 [Google Scholar] [PubMed] [CrossRef]
Tomita T, Bzik DJ, Ma YF, Fox BA, Markillie LM et al. (2013). The Toxoplasma gondii cyst wall protein CST1 is critical for cyst wall integrity and promotes bradyzoite persistence. PLoS Pathogens 9: 1–15. https://doi.org/10.1371/journal.ppat.1003823 [Google Scholar] [PubMed] [CrossRef]
Torpier G, Charif H, Darcy F, Liu J, Darde ML et al. (1993). Toxoplasma gondii: Differential location of antigens secreted from encysted bradyzoites. Experimental Parasitology 77: 13–22. https://doi.org/10.1006/expr.1993.1056 [Google Scholar] [PubMed] [CrossRef]
Tosh KW, Mittereder L, Bonne-Annee S, Hieny S, Nutman TB et al. (2016). The IL-12 response of primary human DC and monocytes to Toxoplasma gondii is stimulated by phagocytosis of live parasites rather than host cell invasion. Journal of Immunology 196: 345. https://doi.org/10.4049/JIMMUNOL.1501558 [Google Scholar] [PubMed] [CrossRef]
Travier L, Mondragon R, Dubremetz JF, Musset K, Mondragon M et al. (2008). Functional domains of the Toxoplasma GRA2 protein in the formation of the membranous nanotubular network of the parasitophorous vacuole. International Journal for Parasitology 38: 757–773. https://doi.org/10.1016/j.ijpara.2007.10.010 [Google Scholar] [PubMed] [CrossRef]
Tu V, Ma Y, Tomita T, Sugi T, Mayoral J et al. (2020a). The Toxoplasma gondii cyst wall interactome. mBio 11: e02699–19. https://doi.org/10.1128/MBIO.02699-19 [Google Scholar] [PubMed] [CrossRef]
Tu V, Mayoral J, Sugi T, Tomita T, Han B et al. (2019). Enrichment and proteomic characterization of the cyst wall from in vitro Toxoplasma gondii cysts. mBio 10: e00469–19. https://doi.org/10.1128/MBIO.00469-19 [Google Scholar] [PubMed] [CrossRef]
Tu V, Mayoral J, Yakubu RR, Tomita T, Sugi T et al. (2020b). MAG2, a Toxoplasma gondii bradyzoite stage-specific cyst matrix protein. mSphere 5. [Google Scholar]
Turetzky JM, Chu DK, Hajagos BE, Bradley PJ (2010). Processing and secretion of ROP13: A unique Toxoplasma effector protein. International Journal for Parasitology 40: 1037–1044. https://doi.org/10.1016/J.IJPARA.2010.02.014 [Google Scholar] [PubMed] [CrossRef]
Velásquez ZD, Conejeros I, Larrazabal C, Kerner K, Hermosilla C et al. (2019). Toxoplasma gondii-induced host cellular cell cycle dysregulation is linked to chromosome missegregation and cytokinesis failure in primary endothelial host cells. Science Reports 9: 12496. https://doi.org/10.1038/S41598-019-48961-0 [Google Scholar] [PubMed] [CrossRef]
Virreira Winter S, Niedelman W, Jensen KD, Rosowski EE, Julien L et al. (2011). Determinants of GBP recruitment to Toxoplasma gondii vacuoles and the parasitic factors that control it. PLoS One 6: e24434. https://doi.org/10.1371/JOURNAL.PONE.0024434 [Google Scholar] [PubMed] [CrossRef]
Walker ME, Hjort EE, Smith SS, Tripathi A, Hornick JE et al. (2008). Toxoplasma gondii actively remodels the microtubule network in host cells. Microbes and Infection 10: 1440–1449. https://doi.org/10.1016/J.MICINF.2008.08.014 [Google Scholar] [PubMed] [CrossRef]
Wang Y, Cirelli KM, Barros PDC, Sangaré LO, Butty V et al. (2019). Three Toxoplasma gondii dense granule proteins are required for induction of lewis rat macrophage pyroptosis. mBio 10: e02388–18. https://doi.org/10.1128/MBIO.02388-18 [Google Scholar] [PubMed] [CrossRef]
Wang JL, Li TT, Elsheikha HM, Chen K, Zhu WN et al. (2017). Functional characterization of rhoptry kinome in the virulent Toxoplasma gondii RH strain. Frontiers in Microbiology 8: 84. https://doi.org/10.3389/FMICB.2017.00084 [Google Scholar] [PubMed] [CrossRef]
Wang Y, Weiss LM, Orlofsky A (2010). Coordinate control of host centrosome position, organelle distribution, and migratory response by Toxoplasma gondii via host mTORC2. The Journal of Biological Chemistry 285: 15611–15618. https://doi.org/10.1074/JBC.M109.095778 [Google Scholar] [PubMed] [CrossRef]
Williams M, Kim K (2014). From membranes to organelles: Emerging roles for dynamin-like proteins in diverse cellular processes. European Journal of Cell Biology 93: 267–277. https://doi.org/10.1016/J.EJCB.2014.05.002 [Google Scholar] [PubMed] [CrossRef]
Witola WH, Bauman B, McHugh M, Matthews K (2014). Silencing of GRA10 protein expression inhibits Toxoplasma gondii intracellular growth and development. Parasitology International 63: 651–658. https://doi.org/10.1016/j.parint.2014.05.001 [Google Scholar] [PubMed] [CrossRef]
Yamamoto M, Ma JS, Mueller C, Kamiyama N, Saiga H et al. (2011). ATF6beta is a host cellular target of the Toxoplasma gondii virulence factor ROP18. The Journal of Experimental Medicine 208: 1533–1546. https://doi.org/10.1084/JEM.20101660 [Google Scholar] [PubMed] [CrossRef]
Yamamoto M, Standley DM, Takashima S, Saiga H, Okuyama M, Kayama H et al. (2009). A single polymorphic amino acid on Toxoplasma gondii kinase ROP16 determines the direct and strain-specific activation of Stat3. The Journal of Experimental Medicine 206: 2747–2760. https://doi.org/10.1084/JEM.20091703 [Google Scholar] [PubMed] [CrossRef]
Yang S, Parmley SF (1997). Toxoplasma gondii expresses two distinct lactate dehydrogenase homologous genes during its life cycle in intermediate hosts. Gene 184: 1–12. https://doi.org/10.1016/S0378-1119(96)00566-5 [Google Scholar] [PubMed] [CrossRef]
Yap G, Pesin M, Sher A (2000). Cutting edge: IL-12 is required for the maintenance of IFN-γ production in T cells mediating chronic resistance to the intracellular pathogen, Toxoplasma gondii. Journal of Immunology 165: 628–631. https://doi.org/10.4049/JIMMUNOL.165.2.628 [Google Scholar] [PubMed] [CrossRef]
Yap GS, Sher A (1999a). Effector cells of both nonhemopoietic and hemopoietic origin are required for interferon (IFN)-γ- and tumor necrosis factor (TNF)-α-dependent host resistance to the intracellular pathogen, Toxoplasma gondii. The Journal of Experimental Medicine 189: 1083–1091. [Google Scholar] [PubMed]
Yap GS, Sher A (1999b). Cell-mediated immunity to Toxoplasma gondii: initiation, regulation and effector function. Immunobiology 201: 240–247. https://doi.org/10.1016/S0171-2985(99)80064-3 [Google Scholar] [PubMed] [CrossRef]
Zhang YW, Halonen SK, Ma YF, Wittner M, Weiss LM (2001). Initial characterization of CST1, a Toxoplasma gondii cyst wall glycoprotein. Infection and Immunity 69: 501–507. https://doi.org/10.1128/IAI.69.1.501-507.2001 [Google Scholar] [PubMed] [CrossRef]
Zhao Y, Ferguson DJ, Wilson DC, Howard JC, Sibley LD (2009). Virulent Toxoplasma gondii evade immunity-related GTPase-mediated parasite vacuole disruption within primed macrophages. Journal of Immunol 182: 3775–3781. [Google Scholar]
Zhu L, Qi W, Yang G, Yang Y, Wang Y et al. (2022). Toxoplasma gondii rhoptry protein 7 (ROP7) interacts with NLRP3 and promotes inflammasome hyperactivation in THP-1-derived macrophages. Cells 11: 1630. https://doi.org/10.4049/jimmunol.0804190 [Google Scholar] [CrossRef]
Cite This Article
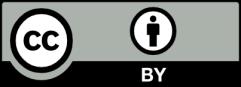
This work is licensed under a Creative Commons Attribution 4.0 International License , which permits unrestricted use, distribution, and reproduction in any medium, provided the original work is properly cited.