Open Access
ARTICLE
The effect of natural products combination on MCF-7 cells exceeds tamoxifen therapeutic dose effects in vitro
1 Department of Biology, Taif University, Taif, 21944, Saudi Arabia
2 Department of Biotechnology, Taif University, Taif, 21944, Saudi Arabia
* Corresponding Author: FAIZAH A. ALMALKI. Email:
(This article belongs to the Special Issue: Herbal Active Ingredients: Potential for the Prevention and Treatment of Cancer)
BIOCELL 2023, 47(4), 891-904. https://doi.org/10.32604/biocell.2023.026556
Received 13 September 2022; Accepted 30 December 2022; Issue published 08 March 2023
Abstract
Cancer remains to be one of the most severe sicknesses globally. Cases have kept rising over the years. Breast cancer (BC), which is among the leading types of cancers and predominantly affects women, is the second leading cause of cancer mortality. Researchers have developed interventions over the years; however, the BC survival rate has not improved since the 1980s. This has created the need for novel drug interventions that would manage and treat BC more effectively. This study focused on using a combination of natural product extracts such as phytoestrogen (Ziziphus jujube) and Tannin nanoparticles (NP99) together, which we have referred to as (Z.NP99) and tamoxifen (Tam) as one of the leading BC drugs since the 70s, in treating BC. The effectiveness of Tam if used alone in the treatment and if combined with Z.NP99 was evaluated using MCF-7 cells in vitro. The findings showed that the combination treatment of Z.NP99 affected the proliferation and viability of MCF-7 cells more than Tam at a 10 μg/mL dose. Moreover, Z.NP99 with Tam stimulated the maximum reduction of MCF-7 proliferation and viability in a time-dependent manner. Furthermore, Tam and Z.NP99 augmented the DNA fragmentation percentage combined with the upregulation of the apoptotic genes. Additionally, the results showed that the apoptotic impact of Z.NP99 and Tam on MCF-7 cells may be intermediated by down-regulating some genes such as Claudin-1 followed by down-regulating mRNA expression of MMP-9, VEGF, and BCL-2 genes of treated cells. Combining Tam with Z.NP99 considerably enhanced the effectiveness of conventional therapy. As a result, this study suggested that the Z.NP99 was ideal for developing effective natural treatments that would improve BC outcomes.Keywords
Breast cancer (BC) remains one of the most severe health issues among women worldwide (Testa et al., 2020). According to the International Agency for Research on Cancer (IARC), BC incidences in females of all ages stood at 47.8 per 100,000 population in 2020, while the age-standardized mortality rate (ASMR) stood at 13.6 per 100,000 population (Globocan, 2020a). Furthermore, IARC Saudi Arabia reported an age-standard incidence rate (ASIR) of 28.8 per 100,000 and an ASMR of 8.9 per 100,000 population (Globocan, 2020b). Likewise, the US cancer statistics cite breast, lung, and colorectal cancers as the most prevalent among women in the country during 2020. They account for 50% of all cancer cases, and among these, 30% of the cases are of BC (Siegel et al., 2020). Cancer prevalence among women presents the urgent need to develop appropriate prevention and therapeutic approaches. This begins with developing robust knowledge about the cellular and molecular origin of the tumor.
Without this information, creating solid preventive frameworks for BC will be nearly impossible. Breast cancer is a highly heterogeneous illness for its histology, epidemiology, and molecular properties (Kennecke et al., 2010). It is clustered into molecular traits, namely hormone receptor activation (estrogen and progesterone receptors), human epidermal growth factor receptor 2 (HER2), and/or BRCA (Testa et al., 2020) BC with estrogen receptor (ER) positivity accounts for approximately 80% of cases (Lumachi et al., 2015). It was logical to use a combined hormone-chemotherapy treatment with the aim of attaining additive and synergistic outcomes. This approach was necessary because BC comprises a mixed population of receptor-positive and receptor-negative cells (Osborne, 1998). Tamoxifen (Tam) (Nolvadex, ICI Pharma, Wilmington, Delaware) hormone-chemotherapy treatment for BC was approved in 1970 (Osborne, 1998; Harper and Walpole, 1966; Klaab et al., 2018). The approval of Tam was linked to its antiestrogenic elements, effectiveness in adjuvant therapy, and its capacity to prevent estrogen receptor-positive (ER+) BC, which is common in over 69% of BC patients.
Nonetheless, utilizing Tam as a therapy also depicts some restrictions on its effectiveness, including stability, solubility, toxicity, delivery to target sites, and possible side effects (Glassman et al., 2017). Tam has numerous side effects; it impairs glucose and lipid metabolism, thereby increasing diabetes incidence (Klöting et al., 2020), causes hypertriglyceridemia, which could lead to acute pancreatitis (Tey et al., 2019), and is linked to ovarian cysts and elevated amounts of estradiol in premenopausal women (Goeminne et al., 2019). Despite its side effects, some patients present resistance to Tam. The resistance occurs in up to 30% of cases, thereby progressing to secondary tumors and eventual mortality over a specific period. Thus, it is critical to apply alternative methods for cancer treatment. One of the most important aspects of cancer therapy is the combination of several treatments to specifically target cancer-inducing or cancer-sustaining pathways to provide the most effective results (Yap et al., 2013; Blagosklonny, 2004). Traditional mono-therapeutic techniques do not distinguish between healthy and cancerous cells, so they ultimately destroy both types of cells. Patients who receive chemotherapy may experience multiple side effects and risks, as well as a significant reduction in immune function as a result of drug effects on bone marrow cells and increased susceptibility to infections (Partridge et al., 2001; LeBaron et al., 1988). Importantly, combination therapy can be toxic if it includes chemotherapeutic agents, but the toxicity is significantly reduced because different pathways are targeted. Therefore, the therapeutic dosages of each drug are reduced because these drugs act synergistically or additively (Albain et al., 2008; Mokhtari et al., 2013). Furthermore, Combination therapies may be able to kill cancer cells while inhibiting their toxic effects on healthy cells at the same time. The possible cause is when one of the drugs in the combination may have antagonistic effects, concerning cytotoxicity, on another drug in normal cells (Blagosklonny, 2005). Thus, Tam is combined with various other natural and medicinal products as extracts, for instance, phytoestrogen or in the form of functionalized nanoparticles.
Plants remain a censorious source in developing medicines that cure different types of illnesses. Scientists remain considerably interested in plants because they contain little toxicity to the tissue, are low-cost, and have fewer side effects concerning the standard chemotherapy treatment (Khan et al., 2019). Currently, at least 60% of anticancer medicines emerge from natural compounds (Mohammed et al., 2019). One of the predominant plants is the Ziziphus jujube, a phytoestrogen belonging to the family Rhamnaceae, generally known as annep, in Saudi Arabia. The plant is rich in biological and phytochemical properties and can cure various diseases, such as ulcers, asthma, and depression. It also has anti-inflammatory, antioxidant, and antimicrobial activities (Batool et al., 2019). Induction of apoptosis using Z. jujube aqueous extract has depicted promising results in killing cervical and breast cancer cells (Pandey and Poonia, 2018).
Fruit methanol extracts have been reported to suppress the proliferation of cancer cells and produce antioxidant effects (Pillai, 2020). Our previous study has demonstrated the positive effects of phytoestrogens on BC treatment through work synergistically with chemotherapeutic drugs like Tam (Klaab et al., 2018).
Nanoparticles are considered smart vehicles to transport across various barriers and increase the interaction of therapeutic agents with BC cells, significantly improving the survival rate and forming an integral part of current BC treatment (Gao et al., 2020). Currently, researchers are exploring the development of a natural compound that will model nanoparticles to augment bioavailability and lessen the dose necessary to attain a therapeutic effect and the ability to target particular tissues or organs (Rizvi and Saleh, 2018).
Polyphenols remain the most important natural product compounds that can be produced as nanoparticles.
Examples include condensed tannin. Previous studies have also shown that tannin extract has antimicrobial, antioxidant, antigenotoxic, and anti-tyrosinase properties (Maisetta et al., 2019; Kwon, 2018). This proves that nanomedicine using natural products contributes to developing more efficient modalities for cancer cure and prevention.
Our earlier works highlighted the impact of Tam, Z. jujube, and tannin nanoparticles (NP99) on MCF-7 breast cancer cells. One study examined the individual effect of Z. jujube and Tam as well as the combinatory impact (Klaab et al., 2018). More recent work also evaluated the cytotoxic effects of Tam and tannin nanoparticles on MCF-7 cells, individually and jointly (AlMalki et al., 2021).
In this evaluation, Tam, a standard therapeutic agent of ER+ BC, Z. jujube extract, a phytoestrogen, and tannin nanoparticles were used jointly on MCF-7 BC cells in vitro. The study also examined the potential molecular processes through which these agents affect resistance, proliferation, and apoptotic pathways. They all augmented the sensitivity of anticancer drugs.
MCF-7 cell line has been chosen for the present study because this work is extended from our previous investigations that applied MCF-7 cells and used phytoestrogen extracts (Klaab et al., 2018; AlMalki et al., 2021). Additionally, MCF-7’s popularity stems from its excellent hormone sensitivity due to the expression of the estrogen receptor (ER+), making it a crucial model for hormone research (Levenson and Jordan, 1997).
Keeping estrogen receptor expression in cultured cells is particularly challenging, thus leading to a high proportion of ER-negative cell lines rather than ER-positive ones (Novaro et al., 2003).
Penicillin-Streptomycin Solution (10.000 Units/mL penicillin, 10.000 µ/mL streptomycin), Dulbecco’s Modified Eagle Medium (DMEM), 0.25% trypsin with 1 mM EDTA (1 X) and fetal bovine serum (FBS) were obtained from (Gibco by Life Technologies, USA), Trizol reagent (Ambion RNA by Life Technology, USA). Gene expression Master Mix (FIREPol®), phosphate-buffered saline (PBS) (Gibco by Life Technologies, USA), 0.4% Trypan blue (Sigma-Aldrich), and MTT (Bio Basic Inc., Canada). All other chemicals used were of a high grade.
The MCF-7 cell line was originally sourced from American Type Culture Collection. The MCF-7 cells were cultured and plated at 1 × 106 cells/well in 6-well plates containing a complete DMEM culture medium with 10% FBS and 1% penicillin-streptomycin antibiotics and were allowed to attach overnight at 37°C in a humidified atmosphere containing 5% CO2 and 95% air (Walle et al., 2007; Klaab et al., 2018). These cells were treated on day 0, after 24 h in basal medium with a medium containing various treatments (experimental groups), and then 24, 48, and 72-h incubation. The experiments were divided using groups of treatments summarized in Fig. 1.
Figure 1: The diagram shows the treatment groups and experiment design.
Pure tamoxifen drug from EBEWE Pharma®-UK was obtained from King Abdul-Aziz Specialist Hospital, Taif, Saudi Arabia. Tam was dissolved in absolute ethanol to make a stock solution of 10 mg/mL. The stock solution was further diluted in 1.0 mg/mL in 10% ethanol before being added to the culture. The second process was necessary due to the low solubility of tamoxifen in water. The culture gave an ultimate concentration of 10 µg/mL medium (Ichikawa et al., 2008).
Ziziphus jujube extract
The extract of Z. jujube was acquired as capsules, each with 500 mg of pure Jujube fruit extract without any additives. The extract was purchased from Source Naturals, Amazon (GMP, FDA, Registered FA City, USA). Pure Jujube extract was dissolved in warm distilled water for 30 min. It was prepared freshly, just before the treatment (Klaab et al., 2018).
Fifty milliliters of purified water and propylene glycol were heated at 70°C in a 1:1 ratio in a beaker. Ethylenediaminetetraacetic acid (EDTA, 0.5%) and poloxamer 407 (pluronic F-127, 0.5%) were then added to the solution while continuously stirring, and the mixture was cooled to 50°C. It was further cooled to 40°C, and the NP99 was added into the carbopol gel and mixed evenly to create a viable gel. The NP99 was dispersed into the carbopol gel to obtain the final concentration of NP99 at 1.5%, while the ultimate concentration of carbopol was maintained at 2%.
The NP99 powder was diffused in sterilized water to come up with varied concentrations to examine its cytotoxic effect on MCF-7 cells, which showed a half-maximal inhibitory concentration (IC50) value at 18 µg/mL (AlMalki et al., 2021).
Cell proliferation assay
Counting of proliferating cells was done using Trypan blue staining. The living and healthy cells are not colored because they are not able to absorb trypan blue. Consequently, dead cells are visible under a microscope as blue. Following the removal of the culture medium, attached cells were rinsed in PBS. Immediately after trypsinization, centrifuge at 2500 rpm for 7 min at 4°C, dispose of the media, and 1 mL of fresh media was added. Using a microcentrifuge tube, add 10 μL of the cell suspension along with 100 μL of the dye “Trypan blue,” and apply 10–20 μL of the mixture to the Heamocytometer slide then monitored carefully under a microscope (Strober, 2001).
The 3-(4,5-dimethylthiazol-2-yl) 2,5-diphenyl tetrazolium (MTT) colorimetric assay was used to assess the viability and cytotoxicity of MCF-7cells induced after the treatment of Z.NP99 and Tam as it is used to test the activity of mitochondrial dehydrogenase producing purple formazan salt crystals (Mosmann, 1983; Wen et al., 2019). The cells were seeded onto the 96-well microtiter plates and treatments were performed separately and in combination.
After incubation at different time courses (24, 48 & 72 h), the medium was removed, and 40 μL MTT solution (5 mg/mL) was added to each well for 4 h at 37°C with a 5% CO2 incubator. After that, the MTT solution was removed and replaced with 140 μL of dimethyl sulfoxide (DMSO) to solubilize the formed formazan. The absorbance was measured using a plate reader (MR-96A) at a test wavelength of 540 nm. The experiments were done in quadruplicate and are representative of at least three independent experiments (Rasul et al., 2011a; Rasul et al., 2011b). The inhibition of percentage growth was determined using the formula below:
Inhibition%= [A540(control) - A540(treated)]/ A540(control)×100].
Programmed cell death (apoptosis) was evaluated for natural products Z.NP99 and Tam using BioVision’s Quick Apoptotic DNA Ladder Detection Kit (Bio-Vision, Life Science SOURCE™, USA) as indicated in (Klaab et al., 2018; AlMalki et al., 2021). DNA was extracted from MCF-7 cells, and DNA fragments were examined using the Kit. The DNA pellet was dissolved in a 30 μL DNA suspension buffer, and samples were then loaded into an agarose gel of 1.5% containing 0.5 μg/mL ethidium bromide. The DNA bands were examined under ultraviolet radiation and the extent of DNA fragmentation was then evaluated and estimated colorimetrically at 575 nm using Diphenylamine (DPA) according to the methods described by Burton (1956) and Perandones et al. (1993).
The effects of the treatments were examined in terms of the mRNA expression of apoptotic genes such as BCL-2 and Bax.
Claudin-1, vascular endothelial growth factor (VEGF), and matrix metalloproteinase-9 (MMP-9) genes were also investigated. In brief, the total isolated RNA was extracted with the TRIzol reagent (Ambion RNA by Life Technology, USA), and its stability was checked by gel electrophoresis. The isolated RNA was reverse transcribed to generate the first-strand cDNA using 2 μg RNA and reverse transcription reagents (Maxime RT PreMix kit) from iNtRON Biotechnology, Korea. The polymerase chain reaction (PCR) reactions were conducted as described by Sun et al. (2013) using a thermal cycler (PXE 0.5 Thermo) and primer pairs described in Table 1 that were introduced in previous studies, to amplify GAPDH (Livak and Schmittgen, 2001), BCL-2 and Bax (Khodapasand et al., 2015), Claudin-1 (Akasaka et al., 2010), VEGF (Lee et al., 2004), and MMP-9 (Darakhshan et al., 2013). The PCR products were separated in Tris-Borate-EDTA buffer on a 1.5% (w/v) agarose gel and visualized on a UV Trans-illuminator. The DNA bands were then scanned, and signal intensities were quantified under the Gel-Pro software (version 3.1 for Windows 3). The ratio between the target genes-amplification product and the glyceraldehyde 3-phosphate dehydrogenase (GAPDH) gene was calculated to standardize the original variation in sample concentration and endogenous control gene for reaction efficiency (Raben et al., 1996).
All statistical analysis was done using the SPSS11 program.
Duncan’s multiple tests were used to determine the significance of the differences between the treated groups after the One way-ANOVA test (Walter and Duncan, 1969).
The values of the results are displayed using Mean + SE. The result was statistically significant if p < 0.05.
Impact of Z.NP99 and Tam treatments on proliferation and viability percentage of MCF-7 cells
The MCF-7 cells were cultured and treated with specific doses of Z.NP99 combination at IC50 dose (Ziziphus 1 mg/mL and NP99 18 µg/mL) in vitro. The Z.NP99 was mixed with Tam in either a therapeutic dose of 10 µg/mL or 1 µg/mL. After treatment with Z.NP99, the MCF-7 cell growth plummeted after a day, two, and three days (p < 0.05), compared to untreated cells and those treated solely with Tam at the exact dosage of 10 or 1 µg/mL.
The extreme cells growth inhibition occurred in the treatment group of Z.NP99 combined with 10 µg/mL Tam by about 55%, 63%, and 73% after 1, 2, and 3-day, exposure, respectively (Table 2). Furthermore, ZNP99 lessened MCF-7 cell viability in a time-dependent manner (Table 3). Interestingly, Tam at 1 µg/mL when combined with Z.NP99 significantly inhibited the proliferation and viability of MCF-7 cells more than Tam alone at the therapeutic dose, 10 µg/mL (Tables 2 and 3). The most significant cell reduction was about 25.67% for Tam 10 µg/mL with Z.NP99 treatment.
Nonetheless, the peak inhibitions in proliferation and viability proportions were observed after treatment for three days (Tables 2 and 3).
Changes induced in MCF-7 cells treated with Z.NP99 and Tam
As observed above, Z.NP99 and Tam impacted MCF-7 cells’ proliferation and viability in different ways. On the one hand, the growth of untreated MCF-7 cells can be delineated as asymmetrical multilateral fusiform. The cells appeared bright, clear, refractive, and fully stretched, with intact membrane and large nuclei under an inverted microscope, as shown in Figs. 2a and 2b. On the other hand, cells treated with Tam individually (Figs. 2c and 2d), Z.NP99 alone (Figs. 2e and 2f), and in combination with Z.NP99 (Figs. 2g and 2h) depicted considerably reduced cellular crowding, had fragmented nuclei, round shape, shrunken, increased in internal complexity, and showed apoptotic appearance.
Figure 2: Inverted microscope images show changes induced in MCF-7 cells. (a & b) control groups after 24 & 48 h, respectively. The cells appeared bright, clear, refractive, and fully stretched with intact membranes and large nuclei. (c & d) cancer cells treated with tamoxifen (Tam) at a therapeutic dose (10 µg/mL) for 24 & 48 h, respectively. (e & f) treated cells with Z.NP99 for 24 & 48 h, respectively while (g & h) treated cells with the combination of Z.NP99 and Tam (10 µg/mL) for 24 & 48 h, respectively. The cells represent apoptotic characterizations with round shapes, and shrunken and fragmented nuclei. Magnification, X 200.
DNA fragmentation percentage in MCF-7 cells treated with Z.NP99 and Tam
DNA fragmentation was performed on the harvested cells’ DNA treated with Tam at concentrations of 10 & 1 µg/mL, Z.NP99, and the combination of Tam and Z.NP99 for 2 days.
Diphenylamine (DPA) was used to determine the level of DNA fragmentation colorimetrically. DNA profile comparisons on agarose gel electrophoresis were also observed. The migration of fragmented DNA was observed as a smear pattern (Fig. 3a).
Figure 3: DNA fragmentation in MCF-7 cells after different treatments for 48 h. (a) DNA profile on agarose gel electrophoresis illustrates DNA fragmentation which was observed as a smear pattern induced in cancer cells: lane 1 control group, lanes 2 & 3 cells treated with tamoxifen (Tam) at 1 µg/mL and 10 µg/mL, respectively, lane 4 cells treated with Z.NP99, lanes 5 & 6 cells treated with Tam 1 + Z.NP99, and Tam 10 + Z.NP99, respectively. (b) DNA fragmentation is represented in a column chart. Results were obtained from three independent experiments and expressed as mean ± SEM.
The percentage of DNA fragmentation was computed (the rate of fragmented DNA divided by the whole amount of DNA) multiplied by 100, as shown in Table 4. The data showed that DNA fragmentation was observed in MCF-7 cells treated with Tam at the therapeutic dose and/or Z.NP99, but the fragmented DNA became overly dominant after Z.NP99 and Tam in both doses were combined (Fig. 3b).
These observations further supported the apoptotic changes appearing in the microscopic results as mentioned above. The low molecular DNA fragments could be easily detected on the lanes of the combination treatment, and this appearance is a typical characteristic of apoptosis.
Alteration of apoptosis regulating genes expression by Tam and Z.NP99 in MCF-7 cells
Effects on mRNA expression of apoptotic genes (Bax and BCL-2)
The study also sought to ascertain the impact of mRNA expression on apoptotic genetic coding, in particular Bax and BCL-2. Bax is a sturdy proapoptotic gene that leads to cytochrome c release from mitochondria, triggers the caspase pathway, and gives rise to apoptosis.
The Bax and BCL-2 mRNA expression was examined in MCF-7 cells by RT-PCR after each treatment. This was necessary to analyze the effect of Tam, and in combination with Z.NP99, to activate apoptosis in treated MCF-7 cells.
As shown in Table 5 and Fig. 4, 1 µg/mL of Tam treatment-induced trivial Bax transcript level elevation when used discretely.
Figure 4: Photograph and histogram illustrate the effect of different treatments on the relative transcript level of apoptosis genes (Bax and BCL-2) in MCF-7 cells after 48 h. The figure showed that Z.NP99 individually and/or with tamoxifen (Tam) decreased the expression of the anti-apoptotic gene BCl-2 and increased the proapoptotic Bax gene expression. (1) Control group cells were treated with (2) Z.NP99, (3) Tam 1, (4) Tam 10, (5) Tam 1 + Z.NP99, and (6) Tam 10 + Z.NP99. Results were obtained from three independent experiments and expressed as mean ± SEM.
Nonetheless, 10 µg/mL of Tam treatment augmented Bax-mRNA expression at 65.5%, compared to untreated MCF-7 cells. Bax-mRNA elevation expression was 2.8 times higher than untreated MCF-7 cells (cells treated with 10 µg/mL of Tam and Z.NP99 combination). Conversely, Tam lowered BCL-2 mRNA expression by approximately 31.2% compared to untreated cells. The results showed that Z.NP99 could lessen the expression of the anti-apoptotic gene BCL-2, elevate the proapoptotic Bax gene expression, and reduce the BCL-2/Bax ratio, as highlighted in Table 5 and Fig. 4.
Effects on mRNA expression of Claudin-1, VEGF, and MMP-9 genes
The effects of Z.NP99 and Tam demonstrated that they might induce cell apoptosis via modification of other associated genetic coding expressions. This hypothesis was verified by analytically examining Z.NP99 and/or Tam impact on the expression of Claudin-1, VEGF, and MMP-9 genes by semi-quantitative RT-PCR and 2% gel electrophoresis of the products from cells treated with different treatments at the indicated concentration for 2 days. Further, on the one hand, after Tam treatment at 1 µg/mL, the results showed nugatory modifications in the mRNA expression of Claudin-1 (1.011 ± 0.017), VEGF (2.686 ± 0.050), and MMP-9 (1.104 ± 0.031) compared to the untreated cells (1.014 ± 0.058, 2.794 ± 0.057, and 1.118 ± 0.068) respectively as listed in Table 6. On the other hand, when Tam (1 µg/mL) was combined with Z.NP99 a significant reduction in the mRNA expression level of Claudin-1 and VEGF (0.645 ± 0.026, 0.777 ± 0.044, respectively) has observed in MCF-7 treated cells for 48 h. These reductions are less than what was reported for the two genes when cancer cells were treated for two days with Tam at a therapeutic dose (Table 6).
Conversely, Tam (10 µg/mL) and Z.NP99 individually and jointly significantly reduced Claudin-1, VEGF, and MMP-9 mRNA expression in MCF-7 treated cells after 2 days. The combination treatment of the drug and/or both extracts synergistically down-regulated the expression of these genes in MCF-7 treated cells, (Table 6 and Fig. 5).
Figure 5: Photograph and histogram showing the effect of different treatments on the relative transcript level of Claudin-1, VEGF, and MMP-9 genes in MCF-7 cells after 48 h. It is shown that Tam at 1 µg/mL induced insignificant changes in the mRNA expression of these three genes, while the combination of Tam 1 with Z.NP99 reduced the mRNA expression for all genes. Z.NP99 alone and/or with Tam 10 synergistically downregulated the expression of these genes and the maximum effect induced in MCF-7 cells treated with the therapeutic dose of Tam combined with Z.NP99. (1) Control group, then cells treated with (2) Z.NP99, (3) Tam 1, (4) Tam 10, (5) Tam 1 + Z.NP99 and (6) Tam 10 + Z.NP99. Results were obtained from three independent experiments and expressed as mean ± SEM.
The present data demonstrated that the endogenous expression of Claudin-1 mRNA was meaningfully reduced by approximately 50% in MCF-7 cells treated with Z.NP99 extracts compared to untreated MCF-7 cells. The natural products mixture was more potent against MCF-7 cells than the conventional drug in the therapeutic dose as shown in Table 6 and Fig. 5. In addition, the co-treatment of BC cells with Z.NP99 and Tam (10 µg/mL) inhibited Claudin-1, VEGF, and MMP-9 mRNA expression levels more than each one individually.
In our earlier investigations (Klaab et al., 2018; AlMalki et al., 2021), it has been reported that Tam, at the therapeutic dose of 10 µg/mL or at 1 µg/mL treatment, reduced the viability and proliferation percentage of MCF-7 cancer cells in respect to the control group in time and concentration-dependent manner and the maximum reduction was noticed after treatment for three days. The treatment with Z. jujube extract alone induced a significant reduction in the viability of the cell while combination treatment (Z. jujube + 10 µg/mL Tam) was dominant against MCF-7 cells (Klaab et al., 2018).
Treatment with NP99 alone inhibited the growth of MCF-7 cells significantly, while co-treatment of 10 µg/mL Tam and 18 µg/mL of NP99 (IC50) showed more reduction in the growth of the MCF-7 cells (AlMalki et al., 2021).
Previous studies have reported Z. jujube as a valuable herb with extensive medicinal ingredients and have shown a considerable inhibitory impact on various cancer cell proliferation (Huang, 2008; Vahedi et al., 2008; Plastina et al., 2012). Tannin is a complex molecule with a binary performance. Hence, it is a radical scavenger, a chemopreventive agent, and an inducer of oxidative stress under certain conditions (Bouki et al., 2013). Therefore, tannin displays anticarcinogenic characteristics (Katzenellenbogen et al., 2007; Hamiza et al., 2012; Majed et al., 2015), proapoptotic action by producing reactive oxygen species, and the activation of intrinsic apoptotic pathways (Chen et al., 2009; Chang and Wang, 2013; Chen et al., 2014).
Moreover, combining Z. jujube and NP99 individually with Tam could considerably augment the drug’s sensitivity, increase apoptotic activities and show a more prominent reduction in the viability of MCF-7 cells (Klaab et al., 2018; AlMalki et al., 2021). The results of our previous investigations indicate that Tam at 10 µg/mL or 1 µg/mL, when combined with natural extracts, was more sensitive than when used alone (Klaab et al., 2018; AlMalki et al., 2021). This remains an essential observation because drug resistance is the main problem for cancer chemotherapy globally as the search for novel medication with reduced toxicity continues. Additionally, extensive evidence in vitro and in vivo, shows that the anticancer activity of phytoestrogen is due to apoptosis and cell cycle arrest.
The present data showed that combining Tam with Z.NP99 in IC50 values considerably inhibited MCF-7 cell growth and it was more effective and efficient than utilizing Tam alone in treating and managing ER+ BC in vitro. The combination treatment showed acute cytotoxic effects in MCF-7-treated cells compared with the untreated group.
Moreover, morphological investigations on MCF-7 have demonstrated that the treated cells were shrunk and became round in shape, and condensation and aggregation of the nuclear chromatin into dense masses beneath the nuclear membrane appeared after being treated with Tam (10 µg/mL) mixed with Z.NP99 for 48 h. Meanwhile, the features of the apoptotic process (as described above) were more pronounced when MCF-7 cells were treated with the combination treatments of Z.NP99 and/or Tam for 72 h. A considerable percentage of the anticancer drugs originating from plants or synthetic are known to cause DNA damage or suppress its replication by promoting apoptosis. During apoptosis, a specific nuclease, referred to as caspase-activated DNase (CAD) and pre-existed in living cells as an inactive complex, breaks the genomic DNA between nucleosomes and generates DNA fragments. This phenomenon has been used lengthily as a marker in cell death studies via apoptotic cell death (Wyllie, 1980; Nagata, 2000). MCF-7 cells treated with Z.NP99 showed a significant increase in DNA fragmentation percentage associated with modulating the gene expression of pro and anti-apoptotic genes like Bax and BCL-2 at the mRNA level.
During apoptosis, damaged and unnecessary cells are removed from the body. Cancer cells are characterized by resistance to apoptosis, which is caused by the overexpression of proteins that block the process (Hanahan and Weinberg, 2000). To control this programmed cell death, pro-death proteins (like Bax) that cause cell death interact dynamically with pro-survival proteins (BCL-2 family). When the balance between these opposing proteins is shifted, malignant cells may be more susceptible to apoptosis (Chipuk et al., 2010; Dewson and Kluck, 2010). The anti-apoptotic BCL-2 family has been shown to contribute to regulating mitochondria-mediated apoptosis (Chao and Korsmeyer, 1998). These anti-apoptotic proteins contain four conserved BCL-2 homology domains (BH1 to BH4) that form heterodimer complexes with members of the proapoptotic family, such as Bax, to prevent mitochondrial release apoptogenic molecules like cytochrome C to the cytosol (Chao and Korsmeyer, 1998; Oltvai et al., 1993; Mazars et al., 2005). Overexpression of BCL-2 triggers resistance to a variety of apoptotic stimuli, including chemotherapy drugs, in many hematological malignancies and solid tumors (Mazars et al., 2005). Using Z.NP99 combination treatment, the anti- and proapoptotic proteins are selectively and differentially regulated to ameliorate the effects of apoptotic stimuli. As a result, the BCL-2 level in MCF-7 cells decreased in a time-dependent manner, while the level of Bax increased as well, resulting in the induction of apoptosis in MCF-7 cells, possibly through the activation of caspase-9 (Booth et al., 2013; Boucher et al., 2012).
Other related genes, such as Claudin-1, VEGF, and MMP-9 in mRNA levels, have been examined.
Claudins are usually found in the cell membrane and primarily contribute to cell-cell adhesion (Tsukita et al., 2001; Furuse et al., 2002), and the function of Claudin-1 may vary among dissimilar cell types. Claudin-1 is localized to the cell membrane in T47 D cells and in the nucleus and cytoplasm in human colon cancers (Dhawan et al., 2005).
Previous investigations have shown the link between Claudin-1 expression and cellular resistance in tumors (Dhawan et al., 2005; Yoon et al., 2010).
In cancer cells, increased sensitivity to chemotherapy has been attributed to enhanced apoptosis via the extrinsic apoptotic pathway, and Claudin-1 was identified as a regulator of these processes. In a similar way, Claudin-1 may regulate these apoptotic pathways in BC (Zhou et al., 2015). Precisely, cumulative evidence associating the deregulation of Claudins with tumorigenesis suggests that these proteins are essential in multiple cellular processes, including motility and invasion (Dhawan et al., 2005; Agarwal et al., 2005; Oku et al., 2006; Todd et al., 2015).
According to Akasaka et al. (2010), Claudin-1 exerts anti-apoptotic activity and regulates B-catenin and E-cadherin expression and subcellular localization of MCF-7, but not the T47D cells. They also showed that Claudin-1 expression increased in MCF-7 cells treated with 40 μM Tam, which disagrees with our data and may depend on the Tam concentration used in the treatment. Additionally, their results demonstrated that Claudin-1 knockdown with or without Tam did not affect apoptosis-related proteins like Bax, BCL-2, p53, and p21 (Akasaka et al., 2010).
Another gene that we have investigated is VEGF, a key cytokine involved in tumor growth and development.
Various types of tumors grow invasively and noninvasively through angiogenesis, the formation of new blood vessels that supply oxygen, and nutrients to cancer tissues and remove the waste products. This process is largely mediated by the VEGF gene (Ferrara et al., 2003; Kim et al., 1993).
It is known that matrix metalloproteinases (MMPs) are zinc-dependent endopeptidases. The human body expresses at least 23 of the 28 types of MMPs found in vertebrates (Cui et al., 2017). In addition to proteolytic degradation of the extracellular matrix (ECM), alteration of the cell-cell and cell-ECM interactions, migration, and angiogenesis, they play a key role in tumor growth and invasion (Gialeli et al., 2011). Furthermore, they influence the microenvironment during cancer progression (Kessenbrock et al., 2010). Apoptosis and anti-apoptosis can be induced by matrix-degrading enzymes. On the one hand, cancer cells become resistant to apoptosis and chemotherapy due to the proteolytic activity of the MMPs, which inactivates the Fas receptor in the cell surfaces (Strand et al., 2004; Mitsiades et al., 2001). Another possible strategy by which MMPs induce an anti-apoptotic effect is by indirectly activating serine/threonine kinase Akt/protein kinase B through receptors of endothelial growth factors (EGFR) and IGFR signaling cascades (Gialeli et al., 2009; Kulik et al., 1997). On the other hand, MMPs also can induce apoptosis through an indirect mechanism by altering the extracellular matrix composition (Sympson et al., 1994).
Tumor angiogenesis requires MMP-9, which controls the bioavailability of VEGF, the most powerful angiogenic factor. For instance, pancreatic tumors are able to undergo an angiogenic switch when the MMP-9 enzyme releases sequestered VEGF to its receptor, VEGFR2 (Bergers et al., 2000). BCL-2 is produced by the action of VEGF (Saleem et al., 2013). Therefore, VEGF inhibits tumor cell apoptosis with its effects on the expression of BCL-2 and angiogenesis (Pidgeon et al., 2001).
The present study findings also showed that the Z.NP99 combination treatment noticeably contracted VEGF and MMP-9 mRNA expression in MCF-7 treated cells for 48 h. It collectively down-regulated the expression of both genes in MCF-7 treated cells. Besides, Lee et al. (2004) observed a reduction in VEGF mRNA expression in MCF-7 cells treated with Tam (Lee et al., 2004). This confirmed the current study findings that may explain the antagonistic effect of Tam or phytoestrogens on ER+ breast cancer.
Further, Nilsson et al. (2007) showed that estradiol induced a significant decrease in the expression level of this gene and it modulated the MMP-2 and -9, and activity in BC cells. Therefore, the impact of estrogen and antiestrogen on MMPs has led to conflicting findings (Pilips and McFadden, 2004; Lymperatou et al., 2013). Conversely, Darakhshan et al. (2013) reported that a decline in MMP-9 expression was witnessed after treatment with tranilast alone and in the combined treatment with the Tam. This combination treatment can inhibit growth and proliferation, leading to the angiogenesis of MCF-7 cells. These findings agree with the current investigation results (Darakhshan et al., 2013).
Taken together, Z.NP99 affected the expression of the MMP-9 gene and reduced its production in MCF-7-treated cells. The presence of MMP-9 protein manages VEGF bioavailability which controls the expression of the anti-apoptotic, BCL-2, gene. Thus, we hypothesize that when MMP-9 works in the anti-apoptotic pathway, its expression is abolished or reduced by the action of Z.NP99, followed by decrease in VEGF, which downregulates BCL-2 expression and induces apoptosis in the treated cell. According to the current study finding, under the same conditions, the action of Z.NP99 on MCF-7 cells is higher than the effect of the Tam therapeutic dose (10 μg/mL). Consequently, the combination treatment of Tam 10 and Z.NP99 was more effective on cancer cells, as observed from the results.
Interestingly, reducing the Tam dose to 1 μg/mL had an insignificant effect on MCF-7 alone, but when it was combined with Z.NP99, it became more effective against cancer cells and represented the same effect as the therapeutic dose of Tam (10 μg/mL).
Based on the results, Tam alone is ineffective in treating and managing cancer. Instead, combining it with other plant extracts and nanoparticles like Z.NP99 agents is ideal because it minimizes the side effects of Tam by reducing the therapeutic dosage. The combination creates synergistic anticancer activities of the mixture treatment making Tam more effective. Thus, this treatment combination is ideal for BC treatment and management as well as future research in the field of oncology.
Finally, this study has some limitations, such as the investigation of the efficacy of Tam, Z.NP99, and the combination of long-term assays like clonogenic assay for evaluation of anticancer efficacy. It is the method of choice for determining the fate of cells after exposure to ionizing radiation. However, the clonogenic assay can also measure other agents that cause cytotoxicity. Colonies can be formed only by a small proportion of the seeded cells in vitro. Within one to three weeks following treatment, cells are seeded out in appropriate dilutions to form colonies. At least 50 cells are required to constitute a colony. It tests the ability of each cell in the population to undergo “unlimited” division. (Franken et al., 2006). Additionally, several cancer cells expressing ER+ can be applied, such as MDAMB415, T47D, and CAMA1 (Dai et al., 2017). This study can be carried out in vivo using animal models like mice or rats, which will give accurse data.
Tamoxifen has proven to be one of the most influential and widely used hormone-receptor-positive treatments for BC; some take it to prevent BC. The medicine has been accepted broadly because BC tumors have a high affinity for estrogen and progesterone, or even metastasize. Therefore, Tam, being an estrogen receptor regulator, plugs into specific proteins inside BC cells. Once inside the cells, the drug prevents cancer from accessing the hormones necessary for it to grow and spread. However, Tam has been less effective in preventing and treating BC. As a result, this study sought to examine how the drug can be boosted to attain top-level effectiveness in managing BC. The results were promising in BC treatment and further studies in oncology. In this case, nutritional phytochemicals could play a critical role in developing new BC drugs and highlight promising treatment options for the same type of cancer. The novel drugs could present therapeutic benefits by restraining the primary cellular tumorigenesis activities. This study highlighted the importance of herbs in exerting anti-carcinogenic impact due to their capacity to instigate apoptosis. If this is combined with chemotherapy, it will lead to developing a more effective and efficient approach to cancer treatment rather than utilizing a single agent. This research presented considerable dose reduction when natural extracts and commercial drugs were utilized jointly to treat MCF-7 cells in vitro.
To our knowledge, the current work is the first one to show the anticancer properties of Z.NP99 (Z. jujube and Tannin) extracts in human breast cancer cells.
Acknowledgement: The authors thank Dr. Thamer Ahmed F Bouback, Culture Laboratory, Department of Biology, Faculty of Science, King Abdul-Aziz University, Saudi Arabia, for providing of MCF-7 cell line; Prof. Antonio Pizzi, ENSTIB-LERMAB, University of Lorraine, Epinal, 88051, France for preparing Tannine nanoparticles (NP99) and Prof. Soliman Abdulla, Department of Physics, Faculty of Science, Alexandria University, Alexandria, Egypt for providing of NP99. The authors are also grateful to Taif University for the technical support.
Funding Statement: Researchers Supporting Project No. TURSP-2020/274, Taif University, Taif, Saudi Arabia.
Author Contributions: The authors confirm contribution to the paper as follows: study conception and design: A. H., and F. A.; data collection: Z. K.; analysis and interpretation of results: F. A., A. H., and Z. K.; draft manuscript preparation: F. A., Z. K. and A. J., review and editing: A. J. All authors reviewed the results and approved the final version of the manuscript.
Availability of Data and Materials: All data generated or analyzed during this study are included in this published article.
Ethics Approval: Not applicable.
Conflicts of Interest: The authors declare that they have no conflicts of interest to report regarding the present study.
References
Agarwal R, D’Souza T, Morin PJ (2005). Claudin3 and claudin4 expression in ovarian epithelial cells enhances invasion and is associated with increased matrix metalloproteinase2 activity. Cancer Research 65: 7378–7385. https://doi.org/10.1158/0008-5472.CAN-05-1036 [Google Scholar] [PubMed] [CrossRef]
Akasaka H, Sato F, Morohashi S, Wu Y, Liu Y, Kondo J, Odagiri H, Hakamada K, Kijima H (2010). Anti-apoptotic effect of claudin-1 in tamoxifen-treated human breast cancer MCF-7 cells. BMC Cancer 10: 548. https://doi.org/10.1186/1471-2407-10-548 [Google Scholar] [PubMed] [CrossRef]
Albain KS, Nag SM, Calderillo-Ruiz G, Jordaan JP, Llombart AC et al. (2008). Gemcitabine plus Paclitaxel versus Paclitaxel monotherapy in patients with metastatic breast cancer and prior anthracycline treatment. Journal of Clinical Oncology 26: 3950–3957. https://doi.org/10.1200/JCO.2007.11.9362 [Google Scholar] [PubMed] [CrossRef]
AlMalki FA, Hassan AM, Klaab ZM, Abdulla S, Pizzi A (2021). Tannin nanoparticles (NP99) enhances the anticancer effect of tamoxifen on ER+ breast cancer cells. Journal of Renewable Materials 9: 2077–2092. https://doi.org/10.32604/jrm.2021.016173 [Google Scholar] [CrossRef]
Batool M, Afzal S, Afzal K, Ahmed B, Abbas K, Muhammad SA, Qadir MI (2019). SHORTCOMMUNICATION-Anticancer activity of Ziziphus mauritiana roots against humanbreast cancer cell line. Pakistan Journal of Pharmaceutical Sciences 32: 1715–1716 [Google Scholar] [PubMed]
Bergers G, Brekken R, McMahon G, Vu TH, Itoh T et al. (2000). Matrix metalloproteinase-9 triggers the angiogenic switch during carcinogenesis. Nature Cell Biology 2: 737–744. https://doi.org/10.1038/35036374 [Google Scholar] [PubMed] [CrossRef]
Blagosklonny MV (2004). Analysis of FDA approved anticancer drugs reveals the future of cancer therapy. Cell Cycle 3: 1035–1042. https://doi.org/10.4161/cc.3.8.1023 [Google Scholar] [CrossRef]
Blagosklonny MV (2005). Overcoming limitations of natural anticancer drugs by combining with artificial agents. Trends in Pharmacological Sciences 26: 77–81. https://doi.org/10.1016/j.tips.2004.12.002 [Google Scholar] [PubMed] [CrossRef]
Booth BW, Inskeep BD, Shah H, Park JP, Hay EJ, Burg KJL (2013). Tannic acid preferentially targets estrogen receptor-positive breast cancer. International Journal of Breast Cancer 9: 369609. https://doi.org/10.1155/2013/369609 [Google Scholar] [PubMed] [CrossRef]
Boucher D, Blais V, Denault JB (2012). Caspase-7 uses an exosite to promote poly(ADP ribose) polymerase 1 proteolysis. Proceedings of the National Academy of Sciences of the United States of America 109: 5669–5674. https://doi.org/10.1073/pnas.1200934109 [Google Scholar] [PubMed] [CrossRef]
Bouki E, Dimitriadis VK, Kaloyianni M, Dailianis S (2013). Antioxidant and pro-oxidant challenge of tannic acid in mussel hemocytes exposed to cadmium. Marine Environmental Research 85: 13–20. https://doi.org/10.1016/j.marenvres.2012.12.005 [Google Scholar] [PubMed] [CrossRef]
Burton K (1956). A study of the conditions and mechanism of the diphenylamine reaction for the colorimetric estimation of deoxyribonucleic acid. The Biochemical Journal 62: 315–323. https://doi.org/10.1042/bj0620315 [Google Scholar] [PubMed] [CrossRef]
Chao DT, Korsmeyer SJ (1998). BCL-2 family: Regulators of cell death. Annual Review of Immunology 16: 395–419. https://doi.org/10.1146/annurev.immunol.16.1.395 [Google Scholar] [PubMed] [CrossRef]
Chang TL, Wang CH (2013). Combination of quercetin and tannic acid in inhibiting 26S proteasome affects S5a and 20S expression, and accumulation of ubiquitin resulted in apoptosis in cancer chemoprevention. Biological Chemistry 394: 561–575. https://doi.org/10.1515/hsz-2012-0277 [Google Scholar] [PubMed] [CrossRef]
Chen KS, Hsiao YC, Kuo DY, Chou MC, Chu SC, Hsieh YS, Lin TH (2009). Tannic acid-induced apoptosis and -enhanced sensitivity to arsenic trioxide in human leukemia HL-60 cells. Leukemia Research 33: 297–307. https://doi.org/10.1016/j.leukres.2008.08.006 [Google Scholar] [PubMed] [CrossRef]
Chen YC, Chien LH, Huang BM, Chia YC (2014). Toona sinensis (aqueous leaf extracts) induces apoptosis through the generation of ROS and activation of intrinsic apoptotic pathways in human renal carcinoma cells. Journal of Functional Foods 7: 362–372. https://doi.org/10.1016/j.jff.2014.01.024 [Google Scholar] [CrossRef]
Chipuk JE, Moldoveanu T, Llambi F, Parsons MJ, Green DR (2010). The BCL-2 family reunion. Molecular Cell 37: 299–310. https://doi.org/10.1016/j.molcel.2010.01.025 [Google Scholar] [PubMed] [CrossRef]
Cui N, Hu M, Khalil RA (2017). Biochemical and biological attributes of matrix metalloproteinases. Progress in Molecular Biology and Translational Science 147: 1–73. https://doi.org/10.1016/bs.pmbts.2017.02.005 [Google Scholar] [PubMed] [CrossRef]
Dai X, Cheng H, Bai Z, Li J (2017). Breast cancer cell line classification and its relevance with breast tumor subtyping. Journal of Cancer 8: 3131–3141. https://doi.org/10.7150/jca.18457 [Google Scholar] [PubMed] [CrossRef]
Darakhshan S, Bidmeshkipour A, Khazaei M, Ghanbari A (2013). Synergistic effects of tamoxifen and tranilast on VEGF and MMP-9 regulation in cultured human breast cancer cells. Asian Pacific Journal of Cancer Prevention 14: 6869–6874. https://doi.org/10.7314/APJCP.2013.14.11.6869 [Google Scholar] [PubMed] [CrossRef]
Dewson G, Kluck R (2010). Bcl-2 family-regulated apoptosis in health and disease. Cell Health and Cytoskeleton 2: 9–22. https://doi.org/10.2147/CHC.S6228 [Google Scholar] [CrossRef]
Dhawan P, Singh AB, Deane NG, No Y, Shiou SR, Schmidt C, Neff J, Washington MK, Beauchamp RD (2005). Claudin1 regulates cellular transformation and metastatic behavior in colon cancer. The Journal of Clinical Investigation 115: 1765–1776. https://doi.org/10.1172/JCI24543 [Google Scholar] [PubMed] [CrossRef]
Ferrara N, Gerber HP, LeCouter J (2003). The biology of VEGF and its receptors. Nature Medicine 9: 669–676. https://doi.org/10.1038/nm0603-669 [Google Scholar] [PubMed] [CrossRef]
Franken NA, Rodermond HM, Stap J, Haveman J, van Bree C (2006). Clonogenic assay of cells in vitro. Nature Protocols 1: 2315–2319. https://doi.org/10.1038/nprot.2006.339 [Google Scholar] [PubMed] [CrossRef]
Furuse M, Hata M, Furuse K, Yoshida Y, Haratake A, Sugitani Y, Noda T, Kubo A, Tsukita S (2002). Claudin-based tight junctions are crucial for the mammalian epidermal barrier: A lesson from claudin-1-dentificient mice. The Journal of Cell Biology 156: 1099–1111. https://doi.org/10.1083/jcb.200110122 [Google Scholar] [PubMed] [CrossRef]
Gao Y, Tang M, Leung E (2020). Dual or multiple drugs loaded nanoparticles to target breast cancer stem cells. RSC Advances 1: 19089–19105. https://doi.org/10.1039/D0RA02801K [Google Scholar] [PubMed] [CrossRef]
Gialeli CH, Kletsas D, Mavroudis D, Kalofonos HP, Tzanakakis GN, Karamanos NK (2009). Targeting epidermal growth factor receptor in solid tumors: Critical evaluation of the biological importance of therapeutic monoclonal antibodies. Current Medicinal Chemistry 16: 3797–3804. https://doi.org/10.2174/092986709789177984 [Google Scholar] [PubMed] [CrossRef]
Gialeli C, Theocharis AD, Karamanos NK (2011). Roles of matrix metalloproteinases in cancer progression and their pharmacological targeting. The FEBS Journal 278: 16–27. https://doi.org/10.1111/j.1742-4658.2010.07919.x [Google Scholar] [PubMed] [CrossRef]
Glassman D, Hignett S, Rehman S, Linforth R, Salhab M (2017). Adjuvant endocrine therapy for hormone-positive breast cancer, focusing on ovarian suppression and extended treatment: An update. Anticancer Research 37: 5329–5341. https://doi.org/10.21873/anticanres.11959 [Google Scholar] [PubMed] [CrossRef]
Globocan (2020a). Section of cancer information. https://gco.iarc.fr/today/data/factsheets/populations/900-world-fact-sheets.pdf [Google Scholar]
Globocan (2020b). Section of cancer information. https://gco.iarc.fr/today/data/factsheets/populations/682-saudi-arabia-fact-sheets.pdf [Google Scholar]
Goeminne A, Maggen C, Timmerman D, Neven P (2019). P03 management of ovarian cysts in premenopausal women with tamoxifen. International Journal of Gynecological Cancer 29: A54. https://doi.org/10.1136/ijgc-2019-ESGO.68 [Google Scholar] [CrossRef]
Hamiza OO, Rehman MU, Tahir M, Khan R, Khan AQ, Lateef A, Ali F, Sultana S (2012). Amelioration of 1,2 dimethylhydrazine (DMH) induced colon oxidative stress, inflammation and tumor promotion response by tannic acid in wistar rats. Asian Pacific Journal of Cancer Prevention 13: 4393–4402. https://doi.org/10.7314/APJCP.2012.13.9.4393 [Google Scholar] [PubMed] [CrossRef]
Hanahan D, Weinberg RA (2000). The hallmarks of cancer. Cell 100: 57–70. https://doi.org/10.1016/S0092-8674(00)81683-9 [Google Scholar] [PubMed] [CrossRef]
Harper MJK, Walpole AL (1966). Contrasting endocrine activities of eis and trans isomers in a series of substituted triphenylethylenes. Nature 212: 87. https://doi.org/10.1038/212087a0 [Google Scholar] [PubMed] [CrossRef]
Huang X (2008). Mechanism of the growth inhibitory effects of Zizyphus jujuba and green tea extracts in human hepatoma cells (Thesis). Department of Food and Human Health Sciences, Graduate School of Human Life Science, Osaka City University, Japan. [Google Scholar]
Ichikawa A, Ando J, Suda K (2008). G1 arrest and expression of cyclin-dependent kinase inhibitors in tamoxifen-treated MCF-7 human breast cancer cells. Human Cell 21: 28–37. https://doi.org/10.1111/j.1749-0774.2008.00048.x [Google Scholar] [PubMed] [CrossRef]
Katzenellenbogen M, Mizrahi L, Pappo O, Klopstock N, Olam D et al. (2007). Molecular mechanisms of liver carcinogenesis in the mdr2-knockout mice. Molecular Cancer Research 5: 1159–1170. https://doi.org/10.1158/1541-7786.MCR-07-0172 [Google Scholar] [PubMed] [CrossRef]
Kennecke H, Yerushalmi R, Woods R, Cheang MCU, Voduc D, Speers CH, Nielsen TO, Gelmon K (2010). Metastatic behavior of breast cancer subtypes. Journal of Clinical Oncology 28: 3271–3277. https://doi.org/10.1200/JCO.2009.25.9820 [Google Scholar] [PubMed] [CrossRef]
Kessenbrock K, Plaks V, Werb Z (2010). Matrix metalloproteinases: Regulators of the tumor microenvironment. Cell 141: 52–67. https://doi.org/10.1016/j.cell.2010.03.015 [Google Scholar] [PubMed] [CrossRef]
Khan T, Ali M, Khan A, Nisar P, Jan SA, Afridi S, Shinwari ZK (2019). Anticancer plants: A review of the active phytochemicals, applications in animal models, and regulatory aspects. Biomolecules 10: 47. https://doi.org/10.3390/biom10010047 [Google Scholar] [PubMed] [CrossRef]
Khodapasand E, Jafarzadeh N, Farrokhi F, Kamalidehghan B, Houshmand M (2015). Is Bax/Bcl-2 ratio considered as a prognostic marker with age and tumor location in colorectal cancer? Iranian Biomedical Journal 19: 69–75. https://doi.org/10.6091/ibj.1366.2015 [Google Scholar] [PubMed] [CrossRef]
Kim KJ, Li B, Winer J, Armanini M, Gillett N, Phillips HS, Ferrara N (1993). Inhibition of vascular endothelial growth factor-induced angiogenesis suppresses tumour growth in vivo. Nature 362: 841–844. https://doi.org/10.1038/362841a0 [Google Scholar] [PubMed] [CrossRef]
Klaab ZM, AlMalki FA, Hassan AM (2018). Anticancer activities of ziziphus jujuba extract on breast cancer cells in vitro. International Journal of Pharmaceutical Sciences Review and Research 52: 125–132. [Google Scholar]
Klöting N, Kern M, Moruzzi M, Stumvoll M, Blüher M (2020). Tamoxifen treatment causes early hepatic insulin resistance. Acta Diabetologica 57: 495–498. https://doi.org/10.1007/s00592-019-01468-6 [Google Scholar] [PubMed] [CrossRef]
Kulik G, Klippel A, Weber MJ (1997). Antiapoptotic signalling by the insulin-like growth factor I receptor, phosphatidylinositol 3-kinase, and Akt. Molecular and Cellular Biology 17: 1595–1606. https://doi.org/10.1128/MCB.17.3.1595 [Google Scholar] [PubMed] [CrossRef]
Kwon Y (2018). Food-derived polyphenols inhibit the growth of ovarian cancer cells irrespective of their ability to induce antioxidant responses. Heliyon 4: 1–18. https://doi.org/10.1016/j.heliyon.2018.e00753 [Google Scholar] [PubMed] [CrossRef]
Lee JE, Chung KW, Han W, Kim SW, Kim SW, Shin HJ, Bae JY, Noh DY (2004). Effect of estrogen, tamoxifen and epidermal growth factor on the transcriptional regulation of vascular endothelial growth factor in breast cancer cells. Anticancer Research 24: 3961–3964 [Google Scholar] [PubMed]
LeBaron S, Zeltzer LK, LeBaron C, Scott SE, Zeltzer PM (1988). Chemotherapy side effects in pediatric oncology patients: drugs, age, and sex as risk factors. Medical and Pediatric Oncology 16: 263–268. https://doi.org/10.1002/(ISSN)1096-911X [Google Scholar] [CrossRef]
Levenson AS, Jordan VC (1997). MCF-7: The first hormone-responsive breast cancer cell line. Cancer Research 57: 3071–3078 [Google Scholar] [PubMed]
Livak KJ, Schmittgen TD (2001). Analysis of relative gene expression data using real-time quantitative PCR and the 2(-Delta Delta 2−ΔΔCT Method. Methods 25: 402–408. https://doi.org/10.1006/meth.2001.1262 [Google Scholar] [PubMed] [CrossRef]
Lumachi F, Santeufemia DA, Basso SM (2015). Current medical treatment of estrogen receptor-positive breast cancer. World Journal of Biological Chemistry 6: 231–239. https://doi.org/10.4331/wjbc.v6.i3.231 [Google Scholar] [PubMed] [CrossRef]
Lymperatou D, Giannopoulou E, Koutras AK, Kalofonos HP (2013). The exposure of breast cancer cells to fulvestrant and tamoxifen modulates cell migration differently. BioMed Research International 147514: 1–14. https://doi.org/10.1155/2013/147514 [Google Scholar] [PubMed] [CrossRef]
Maisetta G, Batoni G, Caboni P, Esin S, Rinaldi AC, Zucca P (2019). Tannin profile, antioxidant properties, and antimicrobial activity of extracts from two Mediterranean species of parasitic plant Cytinus. Complementary and Alternative Medicine 19: 1–11. https://doi.org/10.1186/s12906-019-2487-7 [Google Scholar] [PubMed] [CrossRef]
Majed F, Rashid S, Khan AQ, Nafees S, Ali N, Ali R, Khan R, Hasan SK, Mehdi SJ, Sultana S (2015). Tannic acid mitigates the DMBA/croton oil-induced skin cancer progression in mice. Molecular and Cellular Biochemistry 399: 217–228. https://doi.org/10.1007/s11010-014-2248-3 [Google Scholar] [PubMed] [CrossRef]
Mazars A, Geneste O, Hickman J (2005). Antagonistes de Bcl-2, thérapies anticancéreuses alternatives [The Bcl-2 family of proteins as drug targets]. Journal de la Societe de Biologie 199: 253–265. https://doi.org/10.1051/jbio:2005027 [Google Scholar] [PubMed] [CrossRef]
Mitsiades N, Yu WH, Poulaki V, Tsokos M, Stamenkovic I (2001). Matrix metalloproteinase-7-mediated cleavage of Fas ligand protects tumor cells from chemotherapeutic drug cytotoxicity. Cancer Research 61: 577–581 [Google Scholar] [PubMed]
Mohammed MSA, Tawfik MSH, Ibrahim AEG (2019). Influence of two extraction methods on essential oils of some Apiaceae family plants. Egyptian Pharmaceutical Journal 18: 160–164. https://doi.org/10.4103/epj.epj_25_18 [Google Scholar] [CrossRef]
Mokhtari RB, Kumar S, Islam SS, Yazdanpanah M, Adeli K, Cutz E, Yeger H (2013). Combination of carbonic anhydrase inhibitor, acetazolamide, and sulforaphane, reduces the viability and growth of bronchial carcinoid cell lines. BMC Cancer 13: 378. https://doi.org/10.1186/1471-2407-13-378 [Google Scholar] [PubMed] [CrossRef]
Mosmann T (1983). Rapid colorimetric assay for cellular growth and survival: Application to proliferation and cytotoxicity assays. Journal of Immunological Methods 65: 55–63. https://doi.org/10.1016/0022-1759(83)90303-4 [Google Scholar] [PubMed] [CrossRef]
Nagata S (2000). Apoptotic DNA fragmentation. Experimental Cell Research 256: 12–18. https://doi.org/10.1006/excr.2000.4834 [Google Scholar] [PubMed] [CrossRef]
Nilsson U, Garvin S, Dabrosin C (2007). MMP-2 and MMP-9 activity is regulated by estradiol and tamoxifen in cultured human breast cancer cells. Breast Cancer Research and Treatment 102: 253–261. https://doi.org/10.1007/s10549-006-9335-4 [Google Scholar] [PubMed] [CrossRef]
Novaro V, Roskelley CD, Bissell MJ (2003). Collagen-IV and laminin-1 regulate estrogen receptor alpha expression and function in mouse mammary epithelial cells. Journal of Cell Science 116: 2975–2986. https://doi.org/10.1242/jcs.00523 [Google Scholar] [PubMed] [CrossRef]
Oku N, Sasabe E, Ueta E, Yamamoto T, Osaki T (2006). Tight junction protein claudin1 enhances the invasive activity of oral squamous cell carcinoma cells by promoting cleavage of laminin5 γ2 chain via matrix metalloproteinase (MMP)2 and membranetype MMP1. Cancer Research 66: 5251– 5257. https://doi.org/10.1158/0008-5472.CAN-05-4478 [Google Scholar] [PubMed] [CrossRef]
Oltvai ZN, Milliman CL, Korsmeyer SJ (1993). Bcl-2 heterodimerizes in vivo with a conserved homolog, Bax, that accelerates programmed cell death. Cell 74: 609–619. https://doi.org/10.1016/0092-8674(93)90509-O [Google Scholar] [PubMed] [CrossRef]
Osborne CK (1998). Tamoxifen in the treatment of breast cancer. New England Journal of Medicine 339: 1609–1618. https://doi.org/10.1056/NEJM199811263392207 [Google Scholar] [PubMed] [CrossRef]
Pandey S, Poonia A (2018). Bioactive compounds, medicinal benefits and value added products of ber fruit: A review. Journal of Pharmacognosy and Phytochemistry 7: 1460–1466. [Google Scholar]
Partridge AH, Burstein HJ, Winer EP (2001). Side effects of chemotherapy and combined chemohormonal therapy in women with early-stage breast cancer. JNCI Monographs 30: 135–142. https://doi.org/10.1093/oxfordjournals.jncimonographs.a003451 [Google Scholar] [PubMed] [CrossRef]
Perandones CE, Illera VA, Peckham D, Stunz LL, Ashman RF (1993). Regulation of apoptosis in vitro in mature murine spleen T cells. Journal of Immunology 151: 3521–3529. https://doi.org/10.4049/jimmunol.151.7.3521 [Google Scholar] [CrossRef]
Pidgeon GP, Barr MP, Harmey JH, Foley DA, Bouchier-Hayes DJ (2001). Vascular endothelial growth factor (VEGF) upregulates BCL-2 and inhibits apoptosis in human and murine mammary adenocarcinoma cells. British Journal of Cancer 85: 273–278. https://doi.org/10.1054/bjoc.2001.1876 [Google Scholar] [PubMed] [CrossRef]
Pilips N, McFadden K (2004). Inhibition of transforming growth factor-beta and matrix metalloproteinases by estrogen and prolactin in breast cancer cells. Cancer Letters 206: 63–68. https://doi.org/10.1016/j.canlet.2003.10.019 [Google Scholar] [PubMed] [CrossRef]
Pillai RG (2020). Antiproliferative actions of Ziziphus jujube fruit extract is mediated through alterations in Bcl2-Bax ratio and through the activation of caspases: Anticancer mechanism of Ziziphus jujube Ber extract. Chemical Biology Letters 7: 41–46. [Google Scholar]
Plastina P, Bonofiglio D, Vizza D, Fazio A, Rovito D, Giordano C (2012). Identification of bioactive constituents of Ziziphus jujube fruit extracts exerting antiproliferative and apoptotic effects in human breast cancer cells. Journal of Ethnopharmacology 140: 325–332. https://doi.org/10.1016/j.jep.2012.01.022 [Google Scholar] [PubMed] [CrossRef]
Raben N, Nichols RC, Martiniuk F, Plotz PH (1996). A model of mRNA splicing in adult lysosomal storage disease (glycogenosis type II). Human Molecular Genetics 5: 995–1000. https://doi.org/10.1093/hmg/5.7.995 [Google Scholar] [PubMed] [CrossRef]
Rasul A, Khan M, Yu B, Ma T, Yang H (2011a). Xanthoxyletin, a coumarin induces S phase arrest and apoptosis in human gastric adenocarcinoma SGC-7901 cells. Asian Pacific Journal of Cancer Prevention 12: 1219–1223 [Google Scholar] [PubMed]
Rasul A, Yu B, Yang LF, Ali M, Khan M, Ma T, Yang H (2011b). Induction of mitochondria-mediated apoptosis in human gastric adenocarcinoma SGC-7901 cells by kuraridin and Nor-kurarinone isolated from Sophora flavescens. Asian Pacific Journal of Cancer Prevention 12: 2499–2504 [Google Scholar] [PubMed]
Rizvi SAA, Saleh AM (2018). Applications of nanoparticle systems in drug delivery technology. Saudi Pharmaceutical Journal 26: 64–70. https://doi.org/10.1016/j.jsps.2017.10.012 [Google Scholar] [PubMed] [CrossRef]
Saleem M, Qadir MI, Perveen N, Ahmad B, Saleem U, Irshad T, Ahmad B (2013). Inhibitors of apoptotic proteins: New targets for anticancer therapy. Chemical Biology & Drug Design 82: 243–251. https://doi.org/10.1111/cbdd.12176 [Google Scholar] [PubMed] [CrossRef]
Siegel RL, Miller KD, Jemal A (2020). Cancer statistics 2020. CA: A Cancer Journal for Clinicians 70: 7–30. https://doi.org/10.3322/caac.21590 [Google Scholar] [PubMed] [CrossRef]
Strand S, Vollmer P, van den Abeelen L, Gottfried D, Alla V, Heid H, Kuball J, Theobald M, Galle PR, Strand D (2004). Cleavage of CD95 by matrix metalloproteinase-7 induces apoptosis resistance in tumour cells. Oncogene 23: 3732–3736. https://doi.org/10.1038/sj.onc.1207387 [Google Scholar] [PubMed] [CrossRef]
Strober W (2001). Trypan blue exclusion test of cell viability. Current Protocols in Immunology. 21: A.3B.1–A.3B.2. https://doi.org/10.1002/0471142735.ima03bs21 [Google Scholar] [PubMed] [CrossRef]
Sun YF, Song CK, Viernstein H, Unger F, Liang ZS (2013). Apoptosis of human breast cancer cells induced by microencapsulated betulinic acid from sour jujube fruits through the mitochondria transduction pathway. Food Chemistry 138: 1998–2007. https://doi.org/10.1016/j.foodchem.2012.10.079 [Google Scholar] [PubMed] [CrossRef]
Sympson CJ, Talhouk RS, Alexander CM, Chin JR, Clift SM, Bissell MJ, Werb Z (1994). Targeted expression of stromelysin-1 in mammary gland provides evidence for a role of proteinases in branching morphogenesis and the requirement for an intact basement membrane for tissue-specific gene expression. The Journal of Cell Biology 125: 681–693. https://doi.org/10.1083/jcb.125.3.681 [Google Scholar] [PubMed] [CrossRef]
Testa U, Castelli G, Pelosi E (2020). Breast cancer: A molecularly heterogenous disease needing subtype-specific treatments. Medical Sciences 8: 18. https://doi.org/10.3390/medsci8010018 [Google Scholar] [PubMed] [CrossRef]
Tey TT, Maung AC, Lim KW, Hsiang JC (2019). Acute pancreatitis caused by tamoxifen-induced severe hypertriglyceridemia after 4 years of tamoxifen use. ACG Case Reports Journal 6: e00025. https://doi.org/10.14309/crj.0000000000000025 [Google Scholar] [PubMed] [CrossRef]
Todd MC, Petty HM, King JM, Piana Marshall BN, Sheller RA, Cuevas ME (2015). Overexpression and delocalization of claudin-3 protein in MCF-7 and MDA-MB-415 breast cancer cell lines. Oncology Letters 10: 156–162. https://doi.org/10.3892/ol.2015.3160 [Google Scholar] [PubMed] [CrossRef]
Tsukita S, Furuse M, Itoh M (2001). Multifunctional strands in tight junctions. Nature Reviews. Molecular Cell Biology 2: 285–293. https://doi.org/10.1038/35067088 [Google Scholar] [PubMed] [CrossRef]
Vahedi F, Fathi Najafi M, Bozari K (2008). Evaluation of inhibitory effect and apoptosis induction of Zyzyphus Jujube on tumor cell lines, an in vitro preliminary study. Cytotechnology 56: 105–111. https://doi.org/10.1007/s10616-008-9131-6 [Google Scholar] [PubMed] [CrossRef]
Walle T, Ta N, Kawamori T, Wen X, Tsuji PA, Walle UK (2007). Cancer chemopreventive properties of orally bioavailable flavonoids--methylated versus unmethylated flavones. Biochemical Pharmacology 73: 1288–1296. https://doi.org/10.1016/j.bcp.2006.12.028 [Google Scholar] [PubMed] [CrossRef]
Walter RA, Duncan DB (1969). A bayes rule for the symmetric multiple comparison problems. Journal of the American Statistical Association 64: 1484–1503. https://doi.org/10.1080/01621459.1969.10501073 [Google Scholar] [CrossRef]
Wen Q, Luo K, Huang H, Liao W, Yang H (2019). Xanthoxyletin inhibits proliferation of human oral squamous carcinoma cells and induces apoptosis, autophagy, and cell cycle arrest by modulation of the MEK/ERK signaling pathway. Medical Science Monitor 25: 8025–8033. https://doi.org/10.12659/MSM.911697 [Google Scholar] [PubMed] [CrossRef]
Wyllie AH (1980). Glucocorticoid-induced thymocyte apoptosis is associated with endogenous endonuclease activation. Nature 284: 555–556. https://doi.org/10.1038/284555a0 [Google Scholar] [PubMed] [CrossRef]
Yap TA, Omlin A, de Bono JS (2013). Development of therapeutic combinations targeting major cancer signaling pathways. Journal of Clinical Oncology 31: 1592–1605. https://doi.org/10.1200/JCO.2011.37.6418 [Google Scholar] [PubMed] [CrossRef]
Yoon CH, Kim MJ, Park MJ, Park IC, Hwang SG, An S, Choi YH, Yoon G, Lee SJ (2010). Claudin-1 acts through c-ablprotein kinase cdelta (pkcdelta) signaling and has a causal role in the acquisition of invasive capacity in human liver cells. The Journal of Biological Chemistry 285: 226–233. https://doi.org/10.1074/jbc.M109.054189 [Google Scholar] [PubMed] [CrossRef]
Zhou B, Blanchard A, Wang N, Ma X, Han J, Schroedter I, Leygue E, Myal Y (2015). Claudin 1 promotes migration and increases sensitivity to tamoxifen and anticancer drugs in luminal-like human breast cancer cells MCF7. Cancer Investigation 33: 429–439. https://doi.org/10.3109/07357907.2015.1060996 [Google Scholar] [CrossRef]
Cite This Article
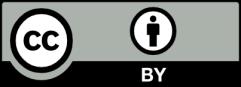
This work is licensed under a Creative Commons Attribution 4.0 International License , which permits unrestricted use, distribution, and reproduction in any medium, provided the original work is properly cited.