Open Access
REVIEW
Understanding cell-extracellular matrix interactions for topology-guided tissue regeneration
1 Department of Biosystems Engineering, Kangwon National University, Chuncheon, 24341, Korea
2 Interdisciplinary Program in Smart Agriculture, Kangwon National University, Chuncheon, 24341, Korea
* Corresponding Author: KI-TAEK LIM. Email:
(This article belongs to the Special Issue: Cell-Based Regenerative Therapies)
BIOCELL 2023, 47(4), 789-808. https://doi.org/10.32604/biocell.2023.026217
Received 24 August 2022; Accepted 15 November 2022; Issue published 08 March 2023
Abstract
Tissues are made up of cells and the extracellular matrix (ECM) which surrounds them. These cells and tissues are actively adaptable to enduring significant stress that occurs in daily life. This astonishing mechanical stress develops due to the interaction between the live cells and the non-living ECM. Cells in the matrix microenvironment can sense the signals and forces produced and initiate a signaling cascade that plays a crucial role in the body’s normal functioning and influences various properties of the native cells, including growth, proliferation, and differentiation. However, the matrix’s characteristic features also impact the repair and regeneration of the damaged tissues. The current study reviewed how the cell-ECM interaction regulates cellular behavior and physicochemical properties. Herein, we have described the response of cells to mechanical stresses, the importance of substrate stiffness and geometry in tissue regeneration, and the development of scaffolds to mimic the nature of native ECM in 3D for tissue engineering applications has also been discussed. Finally, the study summarizes the conclusions and promising prospects based on the cell-ECM interplay.Keywords
Multicellular organisms like humans comprise billions of cells, each serving a specific function in the body’s normal functioning. The interaction between cell to cell and cell to the matrix is crucial to acquire any function. Cells must communicate with the surrounding environment to feed, proliferate, and differentiate. To accomplish this, they should be able to recognize the characteristics of the environment they are living in. Cells can sense the chemicals in their surroundings and respond to them in continuation of the various tasks in the body. During growth and development, cells experience many physical changes such as shear, stretch, and compression. For example, while walking or running, cells in the musculoskeletal system confront mechanical forces, and such cells are referred to as mechanosensitive cells (Evans and Gentleman, 2014; Schwander et al., 2010). An extracellular matrix (ECM) is a three-dimensional network of proteins and other molecules that supports and surrounds the cells inside the body. It is a non-cellular structure that supports tissues and organs. ECM proteins, growth factors, cells, and other substances present in niches help cells exhibit specific structures and functions at nano-, micro-, and macro-scales (Schenke-Layland et al., 2011). It offers various signaling cues that control cell proliferation, differentiation, and adhesion (Huang and Li, 2011). How cells communicate with the ECM and its proteins varies depending on their position. Individual cell types have different anchoring connections between cells and the ECM. Mesenchymal stem cells use adherent junctions to adhere to the encircling ECM, while the epithelial cells are anchored to the ECM via hemidesmosomes. Integrins are cell surface receptors involved in the interaction between cells and ECM. These integrins are heterodimers and serve as transmembrane connectors (Alberts et al., 2002). By structural changes and variations in the binding affinities of integrins, cells may detect and react to the mechanical stresses from the ECM (Puklin-Faucher and Sheetz, 2009). The study of how cells produce and perceive physical and mechanical forces is known as mechanobiology (Ruiz and Chen, 2007). Cell growth, development, homeostasis, and tissue remodeling are, however, influenced by it (Ethier and Simmons, 2007). Various environmental conditions exhibit a shift in cell behavior in 3D culture compared to 2D monolayers. Therefore, it is feasible to control cellular behavior, leading to morphological features that take place in vivo, if the cell microenvironment in vivo can be imitated in vitro (Hussey et al., 2018). Aggregating 3D cells or cell suspension in 3D hydrogels comprised of ECM proteins can induce this imitation (Antoni et al., 2015; Edmondson et al., 2014; Langhans, 2018; Parrish et al., 2018). The “outside-in” and “inside-out” signaling mechanisms that define a cell and ECM are controlled by geometric and molecular requirements. The architecture of cell-cell and cell-matrix, and signaling molecule’s distribution, is influenced by variations in the extracellular environment of the cells (Lee and Vasioukhin, 2008; Morrison and Kimble, 2006; Wodarz and Näthke, 2007).
The cell-matrix communication in tissue repair is becoming increasingly important because mechanobiology-derived scientific advancements in scaffold design methods provide information on the natural and essential cues to facilitate tissue regeneration. This study illustrates the regulation of cellular behavior due to the cell-matrix interactions—impact and importance of substrate characteristics such as stiffness in cell proliferation and differentiation. Furthermore, developing scaffolds with tunable properties to regenerate damaged tissues is also discussed. Fig. 1 represents a schematic overview of the present study.
Figure 1: Schematic representation of cell-matrix interactions and their influence on cell behavior and the development of scaffolds for tissue engineering applications.
Cellular Response to Mechanical Forces
The core of a cell contains a fluidic material packed with various macromolecules, organelles, and components that carry out various processes. The higher-order bundles and meshes are made up of filament networks called the cytoskeleton, which gives cells the potential to withstand extrinsic mechanical stress (Moeendarbary and Harris, 2014). As illustrated in Fig. 2a, microtubules, actin filaments, and a collection of polymers called intermediate filaments are the three primary categories of cytoskeleton polymers. These polymers interact together to regulate the mechanics and morphology of cells. The three cytoskeleton polymers play a crucial role in regulating cell integrity and are arranged into networks that withstand distortion and rearrange in response to external stimuli. When actin filaments and microtubules are polymerized and depolymerized, specific forces are produced that cause alterations in the shape of cells by motor proteins that migrate along the microtubules and actin filament to govern the arrangement of cell constituents (Fletcher and Mullins, 2010). Actin filaments are ~20 μm long, semiflexible, and dynamic, allowing cells to move and alter their shape (Gittes et al., 1993). Single filament stiffness does not significantly impact the actin cytoskeleton’s potential to resist the mechanical force; instead, resistance to mechanical force is a result of a highly organized structure that these filaments create and the associated connections with the polymerizing agents and crosslinkers. The actin cytoskeleton, for instance, creates a thick mesh of 200 nm beneath the apical cell membrane that contributes to the mechanical integrity of the cell, basal fibers bind to the linear bundles, and ECM regulates and maintains stress across the intracellular junction (Moeendarbary and Harris, 2014). Microtubules are rigid polymers and possess challenging assembly kinetics. Microtubules can collapse under the compressive stresses in cells; the length of a microtubule is an estimate of flexibility that improves with rigidity and it corresponds to a persistence length of ~5 mm (Brangwynne et al., 2006). In the interphase of the cell cycle, when cells become ready for division, cells make use of the rigidity by forming radial patterns of microtubules that serve as an important center for the intracellular movement.
Figure 2: (a) A schematic illustration of the cellular cytoskeleton (architecture of cell-cell junction and focal adhesion proteins involved in the cell-ECM interactions) (Septiadi et al., 2018); (b) (i) Fluorescence spectra of B2K G-protein receptor prior to and after 2-min of stimulation by shear stress (ii) Fluorescence degradation dynamics of the receptor proteins in response to the fluid shear stress (Chachisvilis et al., 2006); (c) Effect of stretching on the intracellular concentration of calcium ions (i) Fluo-4 dextran intensity before loading the stretching force (ii) 10 min after loading the stretching force (Munevar et al., 2004).
Actin filaments and microtubules are both polar and possess structural asymmetries in their subunits on a molecular basis. Due to their polarity, both actin filaments and the cytoskeleton function as tracks for motor proteins that travel in a single direction. Dynein and kinesin are motor proteins for the microtubules, while myosin proteins function as motor proteins for the actin filament. These motor proteins are crucial for the organization of the actin cytoskeleton and microtubules and play a significant role in cell mechanics. Microtubules and actin filaments are substantially longer than intermediate filaments; intermediate filaments are categorized as elastic polymers (Gittes et al., 1993; Mücke et al., 2004). They are the least rigid type of cytoskeleton filament and are preferable for resisting tensile stress. Intermediate filaments can form crosslinks with the other filaments, namely, microtubules and actin filaments, via plectin protein (Wiche, 1998). Intermediate filaments can be found in the cytoskeleton and the nuclear envelope, providing structural support to cells (Buehler, 2013). Different adhesion complexes are physically associated with the intermediate filaments. For instance, vimentin intermediate filaments link to integrins directly via connecting to β3 cytoplasmic tail and through bullous pemphigoid antigens 1 and 2 (BPAG) plectin linker proteins by establishing dynamic interactions with plakin repeat motifs (Bhattacharya et al., 2009; Fogl et al., 2016; Kim et al., 2016). In response to external mechanical forces, various cell types generate intermediate filaments; lung epithelial cells generate a network of keratin intermediate filaments that enables the cells to sustain shear stress (Flitney et al., 2009). Intermediate filaments are essential for arranging intracellular organelles and help maintain the cell’s mechanical integrity. Vimentin is a key element influencing the localization of the Golgi complex, mitochondria, nucleus, and endoplasmic reticulum directed by actin and Rac1 (Dupin et al., 2011; Gao and Sztul, 2001; Guo et al., 2013; Matveeva et al., 2015; Nekrasova et al., 2011).
Mechanical forces are essential in balancing the body’s normal functioning and pathophysiology. Various forces, such as compressive, shear, or tensile stress, based upon the cell type, exert their effect on cellular events, for example, cellular differentiation, proliferation, synthesis of proteins, and growth factors. Different types of cells in the body respond to these mechanical forces and are termed mechanosensitive cells, including fibroblasts, tenocytes, chondrocytes, and endothelial cells (Wang and Thampatty, 2008). The evident phenomenon of cell mechanotransduction is not clearly understood, but it is believed that the mechanical stimuli applied to the ECM are transmitted inside the cell via integrin-mediated cell adhesion (Juliano and Haskill, 1993; Maniotis et al., 1997). Integrins are transmembrane receptors with two domains, the ECM and the cytoplasmic domain, that mediate interactions between the cytoskeleton and ECM and are primarily responsible for the mechanotransduction process. Therefore, integrins, ECM, and cytoskeleton are significant players in the study of cell mechanics (Hynes, 1992; Ingber, 1991, 1993). The “tensegrity model” helps to understand the cell mechanics when a mechanical force is initially applied to the plasma membrane and immediately imparted to the cell nucleus via inter-associated cytoskeleton networks and causes activation of several gene expressions (Ingber, 1993; Wang et al., 1993).
In addition to the cytoskeleton and integrins, G proteins are also essential members of mechanobiology. To understand the effect of mechanical stress on G-protein coupled receptors (GPCR), bradykinin B2 (B2K) receptors displayed by bovine aortic endothelial cells are first labeled fluorescently with a yellow fluorescent protein (YFP) and cyan fluorescent proteins (CFP) and subjected to shear stress of ~30 dynes cm2 (for 2 min), leading to observable spectral changes (Fig. 2b(i)). As illustrated in Fig. 2b(ii), the fluorescence decay kinetics of cyan fluorescent protein and yellow fluorescent protein indicates that shear stress causes conformational alterations in the GPCR (Chachisvilis et al., 2006). The concentration of Ca2+ inside the cell also plays a vital role in cell mechanics. Fibroblast cells (NIH3T3) labeled with Fluo-4 were used to examine the effect of stretching forces on cells. The stretching force applied to the fibroblast cells results in an enhanced intensity of the Fluo-4 (Figs. 2c(i) and 2c(ii)), that is proportional to the concentration of intracellular Ca2+; suggesting that applying force to the cells also impacts the calcium concentration inside the cell, which further regulates cellular functions (Munevar et al., 2004).
Influence of mechanical cues on cell adhesion
Membrane deformation leads to membrane adhesion and the activation of G proteins that initiate various cellular events (Gudi et al., 1998; Gudi et al., 1998). The activity of phosphoinositide 3-kinase attributes to the membrane geometry (Hübner et al., 1998). The glycosylphosphatidylinositol (GPI)–anchored proteins potentially function in sensing the mechanical stimuli, as it has been demonstrated that the GPI-linked urokinase plasminogen receptors transduce stresses to the cytoskeleton from the cell surface (Wang et al., 1995). The urokinase plasminogen receptors are physically associated with β-1 integrin to transmit mechanical signals (Wei et al., 1996). Cells are attached to the ECM scaffolds through various receptors called focal adhesion (FA) complexes and to the nearby cells through the junctional complexes. FA complexes include integrins and a range of cytoskeleton-linked proteins (such as vinculin, talin, α-actinin, focal adhesion kinase, and paxillin), which link the F-actin to the integrin’s cytoplasmic tail. These components link and physically connect the ECM to the cytoskeleton (Burridge et al., 1988). As illustrated in Fig. 3a, it is widely acknowledged that integrin, which regulates cell-ECM attachment, can transfer mechanical signals through the membrane. For instance, mechanical forces applied by integrins caused synchronized changes in nuclear and cytoskeleton structure, demonstrating a long-distance force-transferring roadmap from receptor proteins to the nucleus (Maniotis et al., 1997). Blocking the interaction between integrin-ECM inhibits the effect of mechanical forces on the cells (MacKenna et al., 1998; Muller et al., 1997; Salter et al., 1997; Wilson et al., 1995). It is clear from the studies that integrins, which represent a class of transmembrane receptors, are a potential candidate for transferring mechanical stimuli and transmitting instructions encoded in these stimuli into biochemical signals, leading to biological reactions. Platelet-endothelial cell adhesion molecules (PECAM), E-cadherin, and E-selectin are the other adhesion molecules that potentially transfer force through the cell surface to the cytoskeleton (Potard et al., 1997; Wang and Ingber, 1995; Yoshida et al., 1996). The crucial features of cell mechanobiology and its importance in disease and physiology have created tremendous interest in developing techniques for assessing the mechanical characteristics of cells. Cells transmit extracellular or intracellular forces through localized sites at which they adhere to other cells or an extracellular matrix. Various methods have been proposed to examine cell adhesion events by analyzing single cells and cell populations. In general, studies on cell adhesion are grouped into cell attachment and detachment events. Cell adhesion attachment processes rely on the mechanics of cell attachment to the substrate, whereas cell detachment events include applying load to separate the attached cells from the substrate. The examination of the cell adhesion events can be grouped into single cell and cell population analysis (Ahmad Khalili and Ahmad, 2015). The ECM is composed of many proteins and other materials that are required for cellular interactions as listed in Table 1.
Figure 3: (a) A schematic representation of the interaction between the nucleus and cytoskeleton (Patten and Wang, 2021); (b) Designing and Synthesis of scaffolds with different geometry (Bao et al., 2018b); (c) Utilization of stem cell mechanobiology for tissue engineering applications (Vining and Mooney, 2017).
Scaffolds-assisted mechanotransduction
Scaffolds offer the needed support for cell growth and attachment in a 3D milieu, resulting in the development and self-assembly of engineered tissue. The mechanical impact of the scaffolds depends on the designed architecture and characteristics of the scaffold, which include pore size and shape, materials, biodegradability, and elasticity. Scaffolds with varying properties affect cells’ nature and activity (Dado and Levenberg, 2009). The in vivo conditions and the functions of the ECM surrounding enclosed cells can be recreated in a 3D culture system with an extent of complexity that allows cells to behave similarly to their native environment (Naqvi and McNamara, 2020).
To generate the mechanotransduction phenomenon, cells examine substrate stiffness and other environmental stimuli and respond through biochemical components such as the cytoskeleton (Ingber, 2006). There is an interrelationship between the matrix stiffness and cell behavior; the collagen-coated gel of polyacrylamide of stiffness comparable to muscle tissue results in enhanced striations of myotubular actin/myosin (Engler et al., 2004). The stiffness of the matrix has also been shown to control cell differentiation; for example, mesenchymal stem cells, when cultured on polyacrylamide gel with rigidities similar to that of bone, brain, and muscle tissues, caused the cells to differentiate into osteoblasts, neurons, and myoblasts respectively (Engler et al., 2006). Furthermore, it has been demonstrated that the compliance of the substrate may also affect the interactions between nearby endothelial cells. A gentle or soft substrate promotes continuous interactions, whereas a rigid substrate triggers migratory behaviors (Reinhart-King et al., 2008). In a 3D system, the influence of matrix stiffness on stem cell behavior was examined by encapsulating mesenchymal stem cells in poly (ethylene glycol) dimethacrylate of different weight percentages, with which acryloyl-PEG-GRGDS2 was then crosslinked. The cells enclosed in a moderately stiff (11–30 kPa) hydrogel scaffold favored osteogenic differentiation, while the scaffold stiffness of (2.5–5) kPa was preferred for adipogenic differentiation (Huebsch et al., 2010).
Additionally, the mechanical characteristics of PLGA/PLLA scaffolds affect skeletal muscle cell differentiation, organization, and viability; during culture time, stiff scaffolds encourage the differentiation of cells to produce myotubes (Levy-Mishali et al., 2009). Adipogenic differentiation of mesenchymal stem cells (MSCs) occurs when they are enclosed in chemically crosslinked non-degradable hyaluronic acid (HA) stiffness matrices ranging from 4.4–91 kPa, while the degradable HA matrices lead to the osteogenic differentiation of MSCs (Khetan et al., 2013). It is suggested that the differentiation of MSCs can be mediated via the cues offered by crosslinking the hydrogel. Given that scaffold stiffness significantly impacts cell function and tissue integrity, various techniques have been devised to manage scaffold stiffness effectively (Fig. 3c). The range of methods involves adjusting the scaffold’s chemical composition, applying a specified strain, adjusting the architecture, and employing different crosslinking procedures (Cornwell et al., 2007; Hadjipanayi et al., 2009; Karamichos et al., 2007; Lee et al., 2001; Levy-Mishali et al., 2009; Nirmalanandhan et al., 2008). In addition to traditional scaffold manufacturing methods, cell culturing parameters may also modify the stiffness of scaffolds; matrix synthesis and cell-derived molecules produced after culturing are responsible for these findings (Karamichos et al., 2006).
Pores of various sizes and geometries, like rectangles, squares, and cylinders, can alter the function of a cell’s mechanotransduction machinery (Han et al., 2022). Cells can be seeded or injected into a scaffold’s pore structure for delivery to the damaged site. Once inside the pores, the cells adhere to the scaffold surface and subsequently differentiate, proliferate, migrate, and release ECM constituents to aid tissue remodeling (Han et al., 2022). The scaffold’s geometry regulates the matrix by applying a mechanical force gradient to the cells to alter their behavior (Chen et al., 1997). Thus, the ability to recognize geometric properties from meso-to-microscale exists in cells cultured on the surface of the scaffolds (Bao et al., 2018a). For instance, when C2C12 cell lines (myoblast cells) were seeded on fibronectin polydimethylsiloxane with square and rectangular micropatterns, the square geometry showed an increased distribution of histone lysine methyltransferase (SMYD3) compared to the rectangular geometry (Pereira et al., 2020). It has also been demonstrated that the extracellular-related kinase (ERK ½), c-jun N-terminal kinase (JNK), and wingless-type (Wnt) signaling can switch MSCs’ differentiation between adipocytes (round) and osteoblasts (triangle) in a geometry-dependent approach (Kilian et al., 2010). Notably, it is noted that 3D microwells of methacrylate hyaluronic acid (at different volumes of 2800, 3600, and 600 µm3) with triangle, circle, square, and rectangle geometry revealed that among them, only the triangle-shaped microwell enabled cell proliferation and the presence of F-actin and β1 integrin was also observed on both sides of the well (Fig. 3b) (Bao et al., 2018b). In another study, methacrylate hyaluronic acid hydrogel was utilized to create a porous substrate with various geometries (triangular, cuboid, cylinder, and cube) when human-derived mesenchymal stem cells (hBMSCs) were cultured on them instead of cubic and cylinder pores, the cuboid and triangular pores displayed an increased YAP/TAZ nuclear localization (Bao et al., 2017).
Distinctive tissues possess a diverse arrangement of a 3D network of ECM fibrils, for example, isotropic fibers in kidneys and aligned fibers in the tendons (Theocharis et al., 2019; Wang et al., 2018b). As a result, ECM’s 2D and 3D organization can be regulated by biomaterials with varied geometry. Wang et al. (2018b) revealed that an enhanced alignment of ECM on 2D surfaces caused cells to acquire an extended uniaxial shape with enhanced migration and increased Rac activity in fibroblast cells. Consequently, the geometry of a scaffold can be manipulated to regulate the ECM organization and cell migration through a mechanotransduction process (Han et al., 2022). Understanding the critical influence of scaffold geometry on matrix-assisted cellular activity results in developing strategies for manufacturing 3D scaffolds with predetermined geometry (Dado and Levenberg, 2009).
The anisotropy of a material is the characteristic that enables the material to possess different properties when measured in different directions. The successful induction of coordinated cellular proliferation and migration by scaffolds possessing anisotropic macrostructures can promote tissue regeneration (Li et al., 2019; Wang et al., 2020a). Mechanical stretching, ion diffusion, molding, and unidirectional freezing help create scaffolds for various tissue regeneration (Yao et al., 2010; Zhu et al., 2019). Anisotropic scaffolds with directed channels or pores offer cells guiding cues that alter the rate and direction of their growth. According to the research, these structures are beneficial for spinal cord injuries because they encourage orientation, myelination, and outgrowth of axonal and glial cells (Echave et al., 2019; Huang et al., 2020; Rose et al., 2017; Yao et al., 2018; Yu et al., 2020). Antman-Passig and Shefi (2016) fabricated a 3D anisotropic scaffold by incorporating magnetic nanoparticles (mNPs) into the collagen hydrogel and employing an external magnetic field. The neuron cells cultured on the magnetically activated scaffold exhibited typical behavior with high viability and activity (Antman-Passig and Shefi, 2016). Scaffolds with flexible, hydrophilic characteristics can be coupled with topographical signals from fibers to simulate axons’ structure. Aligned fibers allow neurites’ extension, and the scaffold safeguards nerve cells inside the 3D framework, which holds promise for bioinspired nerve regeneration. Thus, anisotropic scaffolds with fibrous structures offer a favorable environment for promoting in vivo axonal growth (Du et al., 2017; Wang et al., 2019; Wu et al., 2019; Zheng et al., 2021). A self-forming multichannel nerve guide conduit (NGC) was fabricated by Wang et al. (2020b) using a shape-memory poly (lactide-co-trimethylene carbonate) polymer. They inserted the NGC into a rat sciatic nerve defect and reported the ability of the conduit to promote nerve regeneration based on the functional evaluation and histological analysis, indicating its ability to repair peripheral nerve abnormalities. Based on these findings, it can be concluded that the anisotropy of a scaffold is an important parameter that enables the unpredictable potential in tissue regeneration (Hu et al., 2022; Zhang et al., 2022).
3D printing methods for scaffolds preparation
Fabrication methods for mimicking the biological activities of cells and tissues include designing a fundamental and structural framework referred to as a scaffold that can support and accommodate the growing cells (Weißenbruch et al., 2022). The most crucial function that scaffolds play is assisting and directing the growth of cells and tissues; in some cases, it also serves as a vehicle for the administration of bioactive chemicals (Hatamzadeh et al., 2016a; Hatamzadeh et al., 2016b). They promote adhesion, migration, and proliferation of the cells, which eventually result in the creation of new tissues; they also influence the characteristics of material transport across the tissues (Tenje et al., 2020). The potential to induce a specific cellular response for the regeneration of new tissues is determined by the designed scaffold’s biological, mechanical, and physiochemical properties (Achberger et al., 2019; Lemma et al., 2019; Spiegel et al., 2020). The two main aspects of fabricating the tissue repairment porous scaffolds are creating interconnected porous structures and maintaining the proper scaffold shape and size (Duan et al., 2014; Pan et al., 2015). Various 3D printing techniques, such as stereolithography, electrospinning (Fig. 5a), and extrusion-based printing, including direct ink writing and fused deposition modeling, allow the synthesis of scaffolds and perform a vital role in tissue regeneration. Some of the printing methods are discussed in the following subsections.
The first additive manufacturing technique to be established and widely used is stereolithography (SLA). The polymerization of bioinks in a 2D layer is controlled using stereolithography rasters that employ a laser beam (Fig. 4a). A UV or visible light source is used to cure a photosensitive hydrogel of polymers such as alginate, hyaluronic acid, and poly (ethylene) glycol (Wang et al., 2021). The process of curing occurs after the deposition of each layer of a substance. When a layer is polymerized, the procedure is rehashed, adding one layer over the previous layer until the whole scaffold is built. A photoinitiator in the liquid polymer resin allows the production of free radicals after absorbing light of a specific wavelength and enables the initiation of polymerization. The production of high-resolution structures is made possible by adjusting several polymerization method parameters, including printing speed, layer thickness, light energy and intensity, and exposure duration (Chen et al., 2018; Hong et al., 2005; Lovell et al., 2001; Watters and Bernhardt, 2018; Yankov and Nikolova, 2017; Zhang et al., 2019).
Figure 4: 3D printing technologies for the development of scaffolds (a) a stereolithography (SLA) printer (Konta et al., 2017); (b) main components of an extrusion-based 3D printer (Jeong et al., 2020); (c) (i) direct ink writing (DIW) printer (Wan et al., 2020); (c) (ii) fused deposition modeling (FDM) printer (Shanmugam et al., 2021).
The SLA technique makes printing various composites possible if the composite possesses a photopolymerizable material or is chemically altered to be used for bone tissue engineering scaffolds (Huang et al., 2017). This technique offers several advantages, such as fast printing speed, high accuracy, smooth surface finish, and high quality. The general printing precision of a typical SLA printer occurs between 1.2–200 µm, depending upon the layer thickness and laser diameter (Ali et al., 2014; Sears et al., 2016). The technique utilizes biomaterials such as composites, ceramics, and biopolymers; composites used in 3D printing methods improve the performance of scaffolds. Lee et al., 2011 used the micro-stereolithography technique to develop a bone-specific poly (propylene fumarate)/diethyl fumarate scaffold and incorporated it with the bone morphogenic protein-2 (BMP-2) loaded microspheres for rat cranial bone. The in vivo results showed enhanced bone formation due to the release of BMP-2 from the scaffold (Lee et al., 2011). Hence, the technology is promising in developing tissue engineering applications structures.
The extrusion-based 3D printing technique was first developed by Crump (1992). This technique is rapidly emerging in the biomedical field. The method employs a broad spectrum of fluids with viscosities varying from 29 mPa s−1 to 6 × 107 mPa s−1 (Ahmad et al., 2019; Crump, 1992). The technique uses a screw device or pneumatic actuator (Fig. 4b) to feed substances via a cartridge or a nozzle for layer-by-layer material deposition. This method allows a high affinity with many materials and attributes a curing step such as light activation or chemical curing. The layer-by-layer deposition of the material can be regulated by actuators that control the arrangement of the nozzle in 3D. Manufacturing complex structures with this technique necessitates a supporting platform as each layer is built above the other (Placone and Engler, 2018). Conventionally, the extrusion method extrudes material by applying pressure. For the printing of tissue-specific scaffolds, the melted polymeric ink is ejected from the nozzle under gradual pressure; the 3D-printed scaffold is generated under the mutual action of the nozzle and lifting table (Duan et al., 2014). To design complex 3D objects, CAD (computer-aided design) software is used to optimize the design. Depending upon the printing temperature, extrusion-based 3D printing methods are divided into two main types: (a) Direct ink writing and (b) Fused deposition modeling (Karyappa and Hashimoto, 2019).
Direct ink writing (DIW) is an extrusion-based 3D printing technique that allows the manufacturing of objects with intricate geometry. In this process, a viscoelastic ink (fluid) is extruded from a printing nozzle to form scaffolds or fibers at room temperature (Fig. 4c(i)), which further accumulates to form a particular pattern as the nozzle moves (Karakurt and Lin, 2020; Li et al., 2021); because of gravity’s influence, the ink is preferentially deposited after wetting the surface. Following a rapid decrease in stress, solvent evaporation, phase change, polymerization, or due to a combined effect, the ink solidifies and builds a structure with the desired properties. DIW is further recognized as Robocasting and is typically classified into two distinct types: droplet and continuous ink extrusion (Lewis et al., 2006; Saadi et al., 2022). The cost-effectiveness and potential to combine various materials in a single-step process in this technique have garnered the attention of many research institutes, and researchers employ DIW constructs for drug encapsulation, soft robotics, and developing touch sensors (Haque et al., 2018; Karakurt and Lin, 2020; Saadi et al., 2022). DIW offers a variety of applications in various areas by monitoring the viscoelastic ink (Karakurt and Lin, 2020).
Fused deposition modeling (FDM)
Fused deposition modeling (FDM) creates 3D structures by using continuous thermoplastic polymer filaments. The main components of a fused deposition modeling setup are a material feed, a gantry, a liquefier print head, and a building surface (Parandoush and Lin, 2017). The operation of FDM can be illustrated by extrusion method, in which the raw material or fed stock is supplied in the form of continuous strips that flow between rollers and get liquified within the liquefier at a temperature higher than its melting point (Dudek, 2013; Varma et al., 2020). The semi-liquid molten filaments are extruded via a nozzle and successively deposited in thin layers on the platform aligned to the XY plane. After the layer deposition, the nozzle head or platform moves along the Z-axis to accurately follow single-layer thickness for subsequent layer assembling (Mandala et al., 2022; Vyavahare et al., 2019). The complete 3D object is produced after a certain period and does not require further processing (Dudek, 2013). This technology, generally with thermoplastics employed as the feed material, generates extremely durable objects in a straightforward one-step procedure appropriate for practical use and provides complete flexibility (Ian Gibson, 2015; Novakova-Marcincinova and Novak-Marcincin, 2012). Fig. 4c(ii) shows the main components of an FDM printer used for creating 3D scaffolds.
Biomimetic scaffolds and tissue engineering
In tissue engineering and regenerative medicines, in addition to the administration of cells to the damaged site by encapsulating them within a scaffold, cells can also be injected or seeded directly inside the pore structure of the scaffold, where the cells attach to the surface of the scaffold and eventually continues the proliferation, differentiation, and secretion of ECM factors to aid in the remodeling and regeneration of the damaged tissues. Therefore, the cells seeded on scaffold surfaces can detect the surface’s geometric properties and translate these cues into mechanical stresses that result in nuclear deformation and changes in gene expression (Bao et al., 2018a; Callens et al., 2020).
Numerous strategies attempt to harness the potential of biomimetic scaffolds to sustain and direct adult stem cell differentiation. The differentiation of mesenchymal stem cells into adipose or osteogenic precursor cells is a mechanosensitive process influenced by the substrate’s stiffness and topography. The bioinspired scaffolds that enhance osteogenic cell differentiation are the fibrous meshwork scaffolds (Figs. 5a(i)–5a(iv)) that can be produced from synthetic material or obtained from biological sources. The biological materials include aligned and decellularized ECM and chitosan/collagen-derived nanofibers; however, polycaprolactone and polyethylene glycol are synthetic materials used to fabricate scaffolds (Abdelmoneim et al., 2020; Azoidis et al., 2017; Ventre et al., 2019; Xie et al., 2016). Table 2 is a list of biomaterials and the effects of exerted mechanical response on different cell types. Furthermore, to improve the rheological properties and enhance the synthesis of mineralized bone matrix, various organic and inorganic components can be effectively incorporated into the scaffold’s backbone (Abdelmoneim et al., 2020; Persson et al., 2018). Recent research developed a highly porous, collagen-functionalized scaffold by employing poly (3,4-ethylene dioxythiophene) polystyrene sulfonate (PEDOT: PSS) as a conductive material. The study revealed that the designed scaffold facilitates osteogenic development and can be a potential candidate for bone tissue regeneration (Iandolo et al., 2020). Electrospun fiber scaffolds comprised of silk fibroin, hyaluronic acid, or polyacrylamide can initiate neurite expansion; their functionalization supports the particular fate of neuronal cells (Farrukh et al., 2017; Hamsici et al., 2017; Seidlits et al., 2019; Sun et al., 2017; Wu et al., 2017). The incorporation of conductive materials such as polyethylene glycol (PEG), poly (3,4-ethylene dioxythiophene), polypyrrole hybrid polymers, and graphene for electrical conduction in the scaffolds leads to the upregulation of glial and neuronal marker proteins (Feig et al., 2021; Rose et al., 2017; Tomaskovic-Crook et al., 2020, 2019; Wang et al., 2018a). Neuronal progenitor cells exhibit a preferential differentiation into the neurons when plated on the scaffolds modified with the laminin-derived peptide sequence (IKVAV) (Silva et al., 2004).
Figure 5: (a) Electrospinning system fabricating micro/nanofibrous scaffold (Jun et al., 2018; Kim et al., 2015a); (b) (i) alkaline phosphatase activity of the cultured stem cells on different scaffolds, (ii–iv) HE staining (v–vi) Von Kossa staining images for the bone formation and calcium deposition analysis (Seyedjafari et al., 2010); (c) SEM images of the chondrocyte proliferation on PHB-chitosan-MWCNT scaffolds (i & ii) day 3 and day 7 (Mohammadalizadeh et al., 2020); (d) muscle wound morphological characteristics followed by 2, 7, 14, and 70 days after transplanting cell loaded fibrin thread based implant (Page et al., 2011).
The most frequent health illnesses are driven by trauma, congenital disease, and aging, the most prevalent cause of cartilage abnormalities. Due to its avascular, lymphatic, and aneural structure, insufficient availability of chondrocytes impedes and obstructs the recovery process at the cartilage defect site. Various types of scaffolds can be manufactured for the regeneration of cartilage tissues based on the availability of polymers and printing methods (Nikbakht et al., 2020). Nanofibrous scaffolds have garnered considerable attention because of their remarkable qualities, including the ability to accommodate labile biomolecules, high volume-to-surface ratio, similarity to the ECM of native tissue, and tunable physical and chemical properties (Farzamfar et al., 2018; Khoshnevisan et al., 2018). Typically, various materials can be used to meet the requirements of an effective scaffold for cartilage tissue regeneration. The blend of synthetic and natural polymers strengthens the physical and mechanical properties of the scaffolds, respectively (Jaymand et al., 2016). Poly (3-hydroxybutyrate) is among the most examined poly-hydroxyalkonate, which is biologically compatible with various cells, but its low hydrophilicity and degradation rate need to be improved for their use in cartilage tissue engineering (Cai et al., 2016). Mohammadalizadeh et al. (2020) developed a multi-walled carbon nanotube (MWNTs) nanofibrous scaffold dispersed in PHB-chitosan that exhibited increased hydrophilicity and three times greater tensile strength. When the chondrocytes were cultured, the scaffolds demonstrated improved growth and cell adhesion properties along with mechanical features similar to the human articular cartilage. 1% MWNTs/PHB-chitosan nanofibrous scaffolds were reported to be the most effective and successful for cartilage tissue regeneration in terms of the structural and biological aspects (Figs. 5c(i) and 5c(ii)) (Mohammadalizadeh et al., 2020). The high mechanical strength and electrical conductivity of the MWNTs make them an adaptive candidate for application in cartilage tissue engineering (Mohammadalizadeh et al., 2020).
Bone injuries and defects pose a serial clinical issue. The risk of musculoskeletal diseases like scoliosis, fracture, osteoporosis, or tumors and diseases like osteoarthritis is rapidly increasing due to the aging global population and longer life expectancy (Agarwal and García, 2015; Roseti et al., 2017). Symptoms like mal-union are frequent seen in patients with severe injuries and restrict the bone from complete recovery (Holzwarth and Ma, 2011). The traditional methods for bone repairment, including bone grafting, hold many limitations due to the lack of availability of donors and the risk of immune rejection. Therefore, the emerging field of tissue engineering offers a novel solution for bone regeneration by developing a potential polymeric scaffold combined with cell and growth factors. The scaffolds are constructed to recreate the natural ECM’s nanofibrous architecture (Zhang and Ma, 2000). Polymers such as poly-l-lactic acid (PLLA), polycaprolactone (PCL), and hydroxyapatite (HA) are employed for designing the scaffolds and are proven to be effective for the treatment of damaged bone tissues. Seyedjafari et al. (2010) developed a hydroxyapatite electrospun PLLA fibrous scaffold seeded with umbilical cord blood (UCB) derived stem cells and inserted into the mice. Following 10 days after the implantation, the results indicated a considerable amount of mineralization with little or no immune reaction to the defective area. The graph in Fig. 5b(i) indicates enhanced mineralization on different scaffolds while Figs. 5b(ii)–5b(vii) shows histological evidence of the bone growth through HE and von Kossa staining (Seyedjafari et al., 2010). Since miRNA can express or suppress the expression of particular genes, it can be employed for tissue regeneration (Peng et al., 2015). Lei et al. (2019) manufactured an injectable hydrogel containing miR-222, aspirin, and silica nanoparticles. After injecting the hydrogel into a bone-defected rat, they revealed that the presence of miR-222 in the hydrogel stimulates neural development in mesenchymal stem cells, facilitating bone restoration and neuron formation (Lei et al., 2019). During the bone repair the effect of miR-26a in enhancing osteoblastic activity can be regulated by targeting Gsk-3 (Glycogen synthase kinase 3) during bone repair (Hao et al., 2017). Besides miRNA and ions, it is ideally possible to enhance the functions of scaffolds by utilizing various biological factors such as fibroblast growth factors (FGFs), bone morphogenetic proteins (BMPs), and transforming growth factors (TGF-β) in order to enhance osteogenesis (Wang et al., 2018c). The most extensively researched factor is bone morphogenetic protein 2 (BMP-2), which can be incorporated into the ECM to establish an osteogenic environment and aid bone formation (Kim et al., 2015b).
With more than 50% of body weight made up of muscle tissue, which regulates the body’s normal functioning, muscles play a crucial role within the body. Nevertheless, as muscles possess a delicate structure, injuries are relatively common. Organ damage and difficulty in movement result from muscular damage (Brack and Rando, 2012; Kwee and Mooney, 2017). A clinical procedure such as surgical restoration is often utilized to treat muscular damage. Consequently, its implementation is severely constrained by low survivability (Garg et al., 2015; Klinkenberg et al., 2013). Integrating biomaterials with a cell-based approach can enhance the therapeutic benefits of cells during muscle regeneration. The fundamental goal of a material is to imitate the original environment and, by associating with the cells, establish a microenvironment that allows the tissues to grow. Page et al. (2011) produced a fibrin fibers scaffold via the micro-thread extrusion method and cultured it with the muscle cells before implanting the scaffold in a significant muscle lesion in the anterior tibialis of mice. The transplanted cell-loaded scaffold promoted the formation of muscle fibers and dramatically lowered fibrosis-related protein expression at the wound site. Fig. 5d depicts the area with regenerating muscles implanted with micro-thread containing less collagen compared to the untreated group (blue arrow) (Page et al., 2011). Patel et al. (2016) constructed a hierarchical carbon scaffold to promote myoblast growth and differentiation. The scaffold was designed in nano and micro-scale geometries. Aligned carbon-fiber mat and random microporous carbon foam were distinct kinds of manufactured scaffolds, and both were grafted with carbon nanotubes to achieve a nanoscale architecture. C2C12 cells exhibited similar proliferative and adhesive properties on both scaffolds. Consequently, the well-aligned fibrous scaffold assists in the conversion of myocytes to myotubes (Patel et al., 2016). In addition, the nanoscale geometry (random or aligned) may alter the surface attributes of the scaffold, hence influencing the adherence and proliferation of myoblasts. However, for myotube production, the synergy between architecture and nanoscale is essential (Dong et al., 2020; Patel et al., 2016).
Conclusions and Future Prospects
The remarks of this review point to the expectation of opening and enabling a new avenue of tissue engineering through mechanotherapy induced by innovative biomaterial platforms. Cells receive a range of mechanical cues from the surrounding environment, and these mechanical signals are widely known for modulating cell physiology and the synthesis of various ECM constituents. Understanding the interaction between cells and matrix sheds light on the development of structures that can mimic the natural environment of ECM and can be a potential parameter for regenerating damaged tissues and organs. Factors including substrate stiffness and architecture are crucial to the study of mechanobiology. Designing scaffolds by regulating the stiffness and surface chemistry helps support cells and tissues’ growth and development. These strategies are essential in investigating and studying cell mechanics that provide a blueprint for developing smart materials in the biomedical field. Despite the versatility and properties offered by 3D scaffolds to simulate cellular microenvironments, several challenges and limitations hinder their application in tissue engineering. For example, the materials used for synthesizing a tissue-specific construct sometimes reduce cell proliferation and trigger the host immune response after implantation; hence, the development of advanced green biomaterials that show enhanced compatibility with the host’s immune system and high degradability needs to be undertaken. The printing resolution of the fabricated scaffold is another challenging parameter; for printing structures with a high resolution, a highly viscous ink is preferable, which damages the cells and restricts their application. Developing a mechanically stable scaffold requires a crosslinking step but, crosslinkers such as glutaraldehyde cause toxicity. Therefore, improved crosslinking methodologies should be applied using crosslinkers such as citric acid and carboxylic acid to strengthen the low mechanical properties. Thus, different types of nature-based polymers and crosslinking agents should be developed to minimize the abovementioned challenges.
Further research is also necessary to analyze the changes in cells and genes during the mechanotransduction process to understand and comprehend the complexity of cell-matrix interplay.
Funding Statement: This research was supported by the Basic Research Program through the National Research Foundation of Korea (NRF), which is financed by the Ministry of Education (Grant Nos. 2018R1A6A1A03025582, 2019R1D1A3A03103828, 2022R1I1A3063302), Korea.
Author Contributions: The authors confirm contribution to the paper as follows: Study conception and design: KTL, AR; Data collection: KTL AR; Data analysis and interpretation: AR, KG, SDD, TVP, DKP; Drafting the article: AR; Critical revision of the article: KTL, SDD; Final approval of the version to be published: KTL.
Availability of Data and Materials: Data sharing is not applicable to this article as no datasets were generated or analyzed during the current study.
Ethics Approval: Not applicable.
Conflicts of Interest: The authors declare that they have no conflicts of interest to report regarding the present study.
References
Abdelmoneim D, Alhamdani GM, Paterson TE, Santocildes Romero ME, Monteiro BJ, Hatton PV, Ortega Asencio I (2020). Bioactive and topographically-modified electrospun membranes for the creation of new bone regeneration models. Processes 8: 1341. https://doi.org/10.3390/pr8111341 [Google Scholar] [CrossRef]
Achberger K, Probst C, Haderspeck J, Bolz S, Rogal J, Chuchuy J, Nikolova M, Cora V, Antkowiak L, Haq W (2019). Merging organoid and organ-on-a-chip technology to generate complex multi-layer tissue models in a human retina-on-a-chip platform. eLife 8: e46188. https://doi.org/10.7554/eLife.46188 [Google Scholar] [PubMed] [CrossRef]
Agarwal R, García AJ (2015). Biomaterial strategies for engineering implants for enhanced osseointegration and bone repair. Advanced Drug Delivery Reviews 94: 53–62. https://doi.org/10.1016/j.addr.2015.03.013 [Google Scholar] [PubMed] [CrossRef]
Ahmad N, Gopinath P, Dutta R (2019). 3D Printing Technology in Nanomedicine. St. Louis, Missouri: Elsevier. https://doi.org/10.1016/B978-0-12-815890-6.00001-3 [Google Scholar] [CrossRef]
Ahmad Khalili A, Ahmad MR (2015). A review of cell adhesion studies for biomedical and biological applications. International Journal of Molecular Sciences 16: 18149–18184. https://doi.org/10.3390/ijms160818149 [Google Scholar] [PubMed] [CrossRef]
Alberts B, Johnson A, Lewis J, Raff M, Roberts K, Walter P (2002). Integrins. In: Molecular Biology of the Cell. 4th edition, New York: Garland Science. [Google Scholar]
Ali M, Pages E, Ducom A, Fontaine A, Guillemot F (2014). Controlling laser-induced jet formation for bioprinting mesenchymal stem cells with high viability and high resolution. Biofabrication 6: 045001. https://doi.org/10.1088/1758-5082/6/4/045001 [Google Scholar] [PubMed] [CrossRef]
Antman-Passig M, Shefi O (2016). Remote magnetic orientation of 3D collagen hydrogels for directed neuronal regeneration. Nano Letters 16: 2567–2573. https://doi.org/10.1021/acs.nanolett.6b00131 [Google Scholar] [PubMed] [CrossRef]
Antoni D, Burckel H, Josset E, Noel G (2015). Three-dimensional cell culture: A breakthrough in vivo. International Journal of Molecular Sciences 16: 5517–5527. https://doi.org/10.3390/ijms16035517 [Google Scholar] [PubMed] [CrossRef]
Azoidis I, Metcalfe J, Reynolds J, Keeton S, Hakki SS, Sheard J, Widera D (2017). Three-dimensional cell culture of human mesenchymal stem cells in nanofibrillar cellulose hydrogels. MRS Communications 7: 458–465. https://doi.org/10.1557/mrc.2017.59 [Google Scholar] [CrossRef]
Bao M, Xie J, Huck WT (2018a). Recent advances in engineering the stem cell microniche in 3D. Advanced Science 5: 1800448. https://doi.org/10.1002/advs.201800448 [Google Scholar] [PubMed] [CrossRef]
Bao M, Xie J, Katoele N, Hu X, Wang B, Piruska A, Huck WT (2018b). Cellular volume and matrix stiffness direct stem cell behavior in a 3D microniche. ACS Applied Materials & Interfaces 11: 1754–1759. https://doi.org/10.1021/acsami.8b19396 [Google Scholar] [PubMed] [CrossRef]
Bao M, Xie J, Piruska A, Huck WT (2017). 3D microniches reveal the importance of cell size and shape. Nature Communications 8: 1–12. https://doi.org/10.1038/s41467-017-02163-2 [Google Scholar] [PubMed] [CrossRef]
Bhattacharya R, Gonzalez AM, DeBiase PJ, Trejo HE, Goldman RD, Flitney FW, Jones JC (2009). Recruitment of vimentin to the cell surface by β3 integrin and plectin mediates adhesion strength. Journal of Cell Science 122: 1390–1400. https://doi.org/10.1242/jcs.043042 [Google Scholar] [PubMed] [CrossRef]
Birchmeier W (1984). Cytoskeleton structure and function. Trends in Biochemical Sciences 9: 192–195. https://doi.org/10.1016/0968-0004(84)90137-3 [Google Scholar] [CrossRef]
Birukov KG, Birukova AA, Dudek SM, Verin AD, Crow MT, Zhan X, DePaola N, Garcia JG (2002). Shear stress-mediated cytoskeletal remodeling and cortactin translocation in pulmonary endothelial cells. American Journal of Respiratory Cell and Molecular Biology 26: 453–464. https://doi.org/10.1165/ajrcmb.26.4.4725 [Google Scholar] [PubMed] [CrossRef]
Brack AS, Rando TA (2012). Tissue-specific stem cells: Lessons from the skeletal muscle satellite cell. Cell Stem Cell 10: 504–514. https://doi.org/10.1016/j.stem.2012.04.001 [Google Scholar] [PubMed] [CrossRef]
Brangwynne CP, MacKintosh FC, Kumar S, Geisse NA, Talbot J, Mahadevan L, Parker KK, Ingber DE, Weitz DA (2006). Microtubules can bear enhanced compressive loads in living cells because of lateral reinforcement. The Journal of Cell Biology 173: 733–741. https://doi.org/10.1083/jcb.200601060 [Google Scholar] [PubMed] [CrossRef]
Bretscher A (1991). Microfilament structure and function in the cortical cytoskeleton. Annual Review of Cell Biology 7: 337–374. https://doi.org/10.1146/annurev.cb.07.110191.002005 [Google Scholar] [PubMed] [CrossRef]
Buehler MJ (2013). Mechanical players—The role of intermediate filaments in cell mechanics and organization. Biophysical Journal 105: 1733–1734. https://doi.org/10.1016/j.bpj.2013.08.050 [Google Scholar] [PubMed] [CrossRef]
Burridge K, Fath K, Kelly T, Nuckolls G, Turner C (1988). Focal adhesions: Transmembrane junctions between the extracellular matrix and the cytoskeleton. Annual Review of Cell Biology 4: 487–525. https://doi.org/10.1146/annurev.cb.04.110188.002415 [Google Scholar] [PubMed] [CrossRef]
Cai Z, Yi X, Yang H, Jia J, Liu Y (2016). Poly (hydroxybutyrate)/cellulose acetate blend nanofiber scaffolds: Preparation, characterization and cytocompatibility. Materials Science and Engineering C 58: 757–767. https://doi.org/10.1016/j.msec.2015.09.048 [Google Scholar] [PubMed] [CrossRef]
Callens SJP, Uyttendaele RJC, Fratila-Apachitei LE, Zadpoor AA (2020). Substrate curvature as a cue to guide spatiotemporal cell and tissue organization. Biomaterials 232: 119739. https://doi.org/10.1016/j.biomaterials.2019.119739 [Google Scholar] [PubMed] [CrossRef]
Caterson B, Lowther DA (1978). Changes in the metabolism of the proteoglycans from sheep articular cartilage in response to mechanical stress. Biochimica et Biophysica Acta (BBA)—General Subjects 540: 412–422. https://doi.org/10.1016/0304-4165(78)90171-X [Google Scholar] [CrossRef]
Chachisvilis M, Zhang YL, Frangos JA (2006). G protein-coupled receptors sense fluid shear stress in endothelial cells. Proceedings of the National Academy of Sciences of the United States of America 103: 15463–15468. https://doi.org/10.1073/pnas.0607224103 [Google Scholar] [PubMed] [CrossRef]
Chen K, Kuang X, Li V, Kang G, Qi HJ (2018). Fabrication of tough epoxy with shape memory effects by UV-assisted direct-ink write printing. Soft Matter 14: 1879–1886. https://doi.org/10.1039/C7SM02362F [Google Scholar] [PubMed] [CrossRef]
Chen CS, Mrksich M, Huang S, Whitesides GM, Ingber DE (1997). Geometric control of cell life and death. Science 276: 1425–1428. https://doi.org/10.1126/science.276.5317.1425 [Google Scholar] [PubMed] [CrossRef]
Chu G, Yuan Z, Zhu C, Zhou P, Wang H, Zhang W, Cai Y, Zhu X, Yang H, Li B (2019). Substrate stiffness- and topography-dependent differentiation of annulus fibrosus-derived stem cells is regulated by Yes-associated protein. Acta Biomaterialia 92: 254–264. https://doi.org/10.1016/j.actbio.2019.05.013 [Google Scholar] [PubMed] [CrossRef]
Ciobanasu C, Faivre B, Le Clainche C (2014). Actomyosin-dependent formation of the mechanosensitive talin-vinculin complex reinforces actin anchoring. Nature Communications 5: 1–10. https://doi.org/10.1038/ncomms4095 [Google Scholar] [PubMed] [CrossRef]
Cornwell KG, Lei P, Andreadis ST, Pins GD (2007). Crosslinking of discrete self-assembled collagen threads: Effects on mechanical strength and cell-matrix interactions. Journal of Biomedical Materials Research Part A 80: 362–371. https://doi.org/10.1002/(ISSN)1552-4965 [Google Scholar] [CrossRef]
Crump SS (1992). Apparatus and method for creating three-dimensional objects (Google Patents). https://patents.google.com/patent/US5121329A/en. [Google Scholar]
Cui H, Liu Y, Deng M, Pang X, Zhang P, Wang X, Chen X, Wei Y (2012). Synthesis of biodegradable and electroactive tetraaniline grafted poly (ester amide) copolymers for bone tissue engineering. Biomacromolecules 13: 2881–2889. https://doi.org/10.1021/bm300897j [Google Scholar] [PubMed] [CrossRef]
Dado D, Levenberg S (2009). Cell–scaffold mechanical interplay within engineered tissue. Seminars in Cell & Developmental Biology 20: 656–664. https://doi.org/10.1016/j.semcdb.2009.02.001 [Google Scholar] [PubMed] [CrossRef]
Dohi T, Padmanabhan J, Akaishi S, Than PA, Terashima M, Matsumoto NN, Ogawa R, Gurtner GC (2019). The interplay of mechanical stress, strain, and stiffness at the keloid periphery correlates with increased caveolin-1/ROCK signaling and scar progression. Plastic and Reconstructive Surgery 144: 58e–67e. https://doi.org/10.1097/PRS.0000000000005717 [Google Scholar] [PubMed] [CrossRef]
Dong R, Ma PX, Guo B (2020). Conductive biomaterials for muscle tissue engineering. Biomaterials 229: 119584. https://doi.org/10.1016/j.biomaterials.2019.119584 [Google Scholar] [PubMed] [CrossRef]
Du J, Liu J, Yao S, Mao H, Peng J et al. (2017). Prompt peripheral nerve regeneration induced by a hierarchically aligned fibrin nanofiber hydrogel. Acta Biomaterialia 55: 296–309. https://doi.org/10.1016/j.actbio.2017.04.010 [Google Scholar] [PubMed] [CrossRef]
Duan P, Pan Z, Cao L, He Y, Wang H, Qu Z, Dong J, Ding J (2014). The effects of pore size in bilayered poly (lactide-co-glycolide) scaffolds on restoring osteochondral defects in rabbits. Journal of Biomedical Materials Research Part A 102: 180–192. https://doi.org/10.1002/jbm.a.34683 [Google Scholar] [PubMed] [CrossRef]
Dudek P (2013). FDM 3D printing technology in manufacturing composite elements. Archives of Metallurgy and Materials 58: 1415–1418. https://doi.org/10.2478/amm-2013-0186 [Google Scholar] [CrossRef]
Dupin I, Sakamoto Y, Etienne-Manneville S (2011). Cytoplasmic intermediate filaments mediate actin-driven positioning of the nucleus. Journal of Cell Science 124: 865–872. https://doi.org/10.1242/jcs.076356 [Google Scholar] [PubMed] [CrossRef]
Echave MC, Domingues RM, Gómez-Florit M, Pedraz JL, Reis RL, Orive G, Gomes ME (2019). Biphasic hydrogels integrating mineralized and anisotropic features for interfacial tissue engineering. ACS Applied Materials & Interfaces 11: 47771–47784. https://doi.org/10.1021/acsami.9b17826 [Google Scholar] [PubMed] [CrossRef]
Edmondson R, Broglie JJ, Adcock AF, Yang L (2014). Three-dimensional cell culture systems and their applications in drug discovery and cell-based biosensors. ASSAY and Drug Development Technologies 12: 207–218. https://doi.org/10.1089/adt.2014.573 [Google Scholar] [PubMed] [CrossRef]
Engler AJ, Griffin MA, Sen S, Bonnemann CG, Sweeney HL, Discher DE (2004). Myotubes differentiate optimally on substrates with tissue-like stiffness: Pathological implications for soft or stiff microenvironments. The Journal of Cell Biology 166: 877–887. https://doi.org/10.1083/jcb.200405004 [Google Scholar] [PubMed] [CrossRef]
Engler AJ, Sen S, Sweeney HL, Discher DE (2006). Matrix elasticity directs stem cell lineage specification. Cell 126: 677–689. https://doi.org/10.1016/j.cell.2006.06.044 [Google Scholar] [PubMed] [CrossRef]
Ethier CR, Simmons CA (2007). Introductory Biomechanics: From Cells to Organisms. New York: Cambridge University Press. [Google Scholar]
Evans ND, Gentleman E (2014). The role of material structure and mechanical properties in cell-matrix interactions. Journal of Materials Chemistry B 2: 2345–2356. https://doi.org/10.1039/c3tb21604g [Google Scholar] [PubMed] [CrossRef]
Farrukh A, Ortega F, Fan W, Marichal N, Paez JI, Berninger B, Del Campo A, Salierno MJ (2017). Bifunctional hydrogels containing the laminin motif IKVAV promote neurogenesis. Stem Cell Reports 9: 1432–1440. https://doi.org/10.1016/j.stemcr.2017.09.002 [Google Scholar] [PubMed] [CrossRef]
Farzamfar S, Naseri-Nosar M, Samadian H, Mahakizadeh S, Tajerian R, Rahmati M, Vaez A, Salehi M (2018). Taurine-loaded poly (ε-caprolactone)/gelatin electrospun mat as a potential wound dressing material: In vitro and in vivo evaluation. Journal of Bioactive and Compatible Polymers 33: 282–294. https://doi.org/10.1177/0883911517737103 [Google Scholar] [CrossRef]
Fazeli N, Arefian E, Irani S, Ardeshirylajimi A, Seyedjafari E (2021). 3D-printed PCL scaffolds coated with nanobioceramics enhance osteogenic differentiation of stem cells. ACS Omega 6: 35284–35296. [Google Scholar] [PubMed]
Feig VR, Santhanam S, McConnell KW, Liu K, Azadian M, Brunel LG, Huang Z, Tran H, George PM, Bao Z (2021). Conducting polymer-based granular hydrogels for injectable 3D cell scaffolds. Advanced Materials Technologies 6: 2100162. https://doi.org/10.1002/admt.202100162 [Google Scholar] [PubMed] [CrossRef]
Fleck CA, Simman R (2010). Modern collagen wound dressings: Function and purpose. The Journal of the American College of Certified Wound Specialists 2: 50–54. https://doi.org/10.1016/j.jcws.2010.12.003 [Google Scholar] [PubMed] [CrossRef]
Fletcher DA, Mullins RD (2010). Cell mechanics and the cytoskeleton. Nature 463: 485–492. https://doi.org/10.1038/nature08908 [Google Scholar] [PubMed] [CrossRef]
Flitney EW, Kuczmarski ER, Adam SA, Goldman RD (2009). Insights into the mechanical properties of epithelial cells: The effects of shear stress on the assembly and remodeling of keratin intermediate filaments. The FASEB Journal 23: 2110–2119. https://doi.org/10.1096/fj.08-124453 [Google Scholar] [PubMed] [CrossRef]
Fogl C, Mohammed F, Al-Jassar C, Jeeves M, Knowles TJ, Rodriguez-Zamora P, White SA, Odintsova E, Overduin M, Chidgey M (2016). Mechanism of intermediate filament recognition by plakin repeat domains revealed by envoplakin targeting of vimentin. Nature Communications 7: 1–11. https://doi.org/10.1038/ncomms10827 [Google Scholar] [PubMed] [CrossRef]
Gao YS, Sztul E (2001). A novel interaction of the Golgi complex with the vimentin intermediate filament cytoskeleton. The Journal of Cell Biology 152: 877–894. https://doi.org/10.1083/jcb.152.5.877 [Google Scholar] [PubMed] [CrossRef]
Gao H, Xiao J, Wei Y, Wang H, Wan H, Liu S (2021). Regulation of myogenic differentiation by topologically microgrooved surfaces for skeletal muscle tissue engineering. ACS Omega 6: 20931–20940. https://doi.org/10.1021/acsomega.1c02347 [Google Scholar] [PubMed] [CrossRef]
Garg K, Corona BT, Walters TJ (2015). Therapeutic strategies for preventing skeletal muscle fibrosis after injury. Frontiers in Pharmacology 6: 87. https://doi.org/10.3389/fphar.2015.00087 [Google Scholar] [PubMed] [CrossRef]
Giancotti FG, Ruoslahti E (1999). Integrin signaling. Science 285: 1028–1033. https://doi.org/10.1126/science.285.5430.1028 [Google Scholar] [PubMed] [CrossRef]
Gittes F, Mickey B, Nettleton J, Howard J (1993). Flexural rigidity of microtubules and actin filaments measured from thermal fluctuations in shape. The Journal of Cell Biology 120: 923–934. https://doi.org/10.1083/jcb.120.4.923 [Google Scholar] [PubMed] [CrossRef]
Gudi S, Lee AA, Clark CB, Frangos JA (1998). Equibiaxial strain and strain rate stimulate early activation of G proteins in cardiac fibroblasts. American Journal of Physiology-Cell Physiology 274: C1424–C1428. https://doi.org/10.1152/ajpcell.1998.274.5.C1424 [Google Scholar] [PubMed] [CrossRef]
Gudi S, Nolan JP, Frangos JA (1998). Modulation of GTPase activity of G proteins by fluid shear stress and phospholipid composition. Proceedings of the National Academy of Sciences of the United States of America 95: 2515–2519. https://doi.org/10.1073/pnas.95.5.2515 [Google Scholar] [PubMed] [CrossRef]
Guo M, Ehrlicher AJ, Mahammad S, Fabich H, Jensen MH, Moore JR, Fredberg JJ, Goldman RD, Weitz DA (2013). The role of vimentin intermediate filaments in cortical and cytoplasmic mechanics. Biophysical Journal 105: 1562–1568. https://doi.org/10.1016/j.bpj.2013.08.037 [Google Scholar] [PubMed] [CrossRef]
Hadjipanayi E, Mudera V, Brown R (2009). Close dependence of fibroblast proliferation on collagen scaffold matrix stiffness. Journal of Tissue Engineering and Regenerative Medicine 3: 77–84. https://doi.org/10.1002/term.136 [Google Scholar] [PubMed] [CrossRef]
Haining AW, Lieberthal TJ, Hernández ADR (2016). Talin: A mechanosensitive molecule in health and disease. The FASEB Journal 30: 2073–2085. https://doi.org/10.1096/fj.201500080R [Google Scholar] [PubMed] [CrossRef]
Hamsici S, Cinar G, Celebioglu A, Uyar T, Tekinay AB, Guler MO (2017). Bioactive peptide functionalized aligned cyclodextrin nanofibers for neurite outgrowth. Journal of Materials Chemistry B 5: 517–524. https://doi.org/10.1039/C6TB02441F [Google Scholar] [PubMed] [CrossRef]
Han P, Gomez GA, Duda GN, Ivanovski S, Poh PS (2022). Scaffold geometry modulation of mechanotransduction and its influence on epigenetics. Acta Biomaterialia. https://doi.org/10.1016/j.actbio.2022.01.020 [Google Scholar] [PubMed] [CrossRef]
Han P, Vaquette C, Abdal-Hay A, Ivanovski S (2021). The mechanosensing and global DNA methylation of human osteoblasts on MEW fibers. Nanomaterials 11: 2943. https://doi.org/10.3390/nano11112943 [Google Scholar] [PubMed] [CrossRef]
Hao Z, Song Z, Huang J, Huang K, Panetta A, Gu Z, Wu J (2017). The scaffold microenvironment for stem cell based bone tissue engineering. Biomaterials Science 5: 1382–1392. https://doi.org/10.1039/C7BM00146K [Google Scholar] [PubMed] [CrossRef]
Haque RI, Chandran O, Lani S, Briand D (2018). Self-powered triboelectric touch sensor made of 3D printed materials. Nano Energy 52: 54–62. https://doi.org/10.1016/j.nanoen.2018.07.038 [Google Scholar] [CrossRef]
Hardy JG, Lee JY, Schmidt CE (2013). Biomimetic conducting polymer-based tissue scaffolds. Current Opinion in Biotechnology 24: 847–854. https://doi.org/10.1016/j.copbio.2013.03.011 [Google Scholar] [PubMed] [CrossRef]
Hatamzadeh M, Najafi-Moghadam P, Baradar-Khoshfetrat A, Jaymand M, Massoumi B (2016a). Novel nanofibrous electrically conductive scaffolds based on poly (ethylene glycol) s-modified polythiophene and poly(ε-caprolactone) for tissue engineering applications. Polymer 107: 177–190. https://doi.org/10.1016/j.polymer.2016.11.012 [Google Scholar] [CrossRef]
Hatamzadeh M, Najafi-Moghadam P, Beygi-Khosrowshahi Y, Massoumi B, Jaymand M (2016b). Electrically conductive nanofibrous scaffolds based on poly (ethylene glycol) s-modified polyaniline and poly(ε-caprolactone) for tissue engineering applications. RSC Advances 6: 105371–105386. https://doi.org/10.1039/C6RA22280C [Google Scholar] [CrossRef]
He Y, Esser P, Schacht V, Bruckner-Tuderman L, Has C (2011). Role of Kindlin-2 in fibroblast functions: Implications for wound healing. Journal of Investigative Dermatology 131: 245–256. https://doi.org/10.1038/jid.2010.273 [Google Scholar] [PubMed] [CrossRef]
Hemmrich K, Salber J, Meersch M, Wiesemann U, Gries T, Pallua N, Klee D (2008). Three-dimensional nonwoven scaffolds from a novel biodegradable poly(ester amide) for tissue engineering applications. Journal of Materials Science: Materials in Medicine 19: 257–267. https://doi.org/10.1007/s10856-006-0048-3 [Google Scholar] [PubMed] [CrossRef]
Holzwarth JM, Ma PX (2011). Biomimetic nanofibrous scaffolds for bone tissue engineering. Biomaterials 32: 9622–9629. https://doi.org/10.1016/j.biomaterials.2011.09.009 [Google Scholar] [PubMed] [CrossRef]
Hong BT, Shin KS, Kim DS (2005). Ultraviolet-curing behavior of an epoxy acrylate resin system. Journal of Applied Polymer Science 98: 1180–1185. https://doi.org/10.1002/(ISSN)1097-4628 [Google Scholar] [CrossRef]
Hoop M, Chen X-Z, Ferrari A, Mushtaq F, Ghazaryan G, Tervoort T, Poulikakos D, Nelson B, Pané S (2017). Ultrasound-mediated piezoelectric differentiation of neuron-like PC12 cells on PVDF membranes. Scientific Reports 7: 1–8. https://doi.org/10.1038/s41598-017-03992-3 [Google Scholar] [PubMed] [CrossRef]
Hu Y, Zhang H, Wei H, Cheng H, Cai J, Chen X, Xia L, Wang H, Chai R (2022). Scaffolds with anisotropic structure for neural tissue engineering. Engineered Regeneration 3: 154–162. https://doi.org/10.1016/j.engreg.2022.04.001 [Google Scholar] [CrossRef]
Huang NF, Li S (2011). Regulation of the matrix microenvironment for stem cell engineering and regenerative medicine. Annals of Biomedical Engineering 39: 1201–1214. https://doi.org/10.1007/s10439-011-0297-2 [Google Scholar] [PubMed] [CrossRef]
Huang L, Wang Y, Zhu M, Wan X, Zhang H, Lei T, Blesch A, Liu S (2020). Anisotropic alginate hydrogels promote axonal growth across chronic spinal cord transections after scar removal. ACS Biomaterials Science & Engineering 6: 2274–2286. https://doi.org/10.1021/acsbiomaterials.9b01802 [Google Scholar] [PubMed] [CrossRef]
Huang Y, Zhang XF, Gao G, Yonezawa T, Cui X (2017). 3D bioprinting and the current applications in tissue engineering. Biotechnology Journal 12: 1600734. https://doi.org/10.1002/biot.201600734 [Google Scholar] [PubMed] [CrossRef]
Huebsch N, Arany P, Mao A, Shvartsman D, Ali OA, Bencherif SA, Rivera-Feliciano J, Mooney DJ (2010). Harnessing traction-mediated manipulation of the cell/matrix interface to control stem-cell fate. Nature Materials 9: 518–526. https://doi.org/10.1038/nmat2732 [Google Scholar] [PubMed] [CrossRef]
Hussey GS, Dziki JL, Badylak SF (2018). Extracellular matrix-based materials for regenerative medicine. Nature Reviews Materials 3: 159–173. https://doi.org/10.1038/s41578-018-0023-x [Google Scholar] [CrossRef]
Hynes RO (1992). Integrins: Versatility, modulation, and signaling in cell adhesion. Cell 69: 11–25. https://doi.org/10.1016/0092-8674(92)90115-S [Google Scholar] [PubMed] [CrossRef]
Hübner S, Couvillon AD, Käs JA, Bankaitis VA, Vegners R, Carpenter CL, Janmey PA (1998). Enhancement of phosphoinositide 3-kinase (PI 3-kinase) activity by membrane curvature and inositol-phospholipid-binding peptides. European Journal of Biochemistry 258: 846–853. https://doi.org/10.1046/j.1432-1327.1998.2580846.x [Google Scholar] [PubMed] [CrossRef]
Ian Gibson IG (2015). Additive Manufacturing Technologies 3D Printing, Rapid Prototyping, and Direct Digital Manufacturing. New York: Springer. [Google Scholar]
Iandolo D, Sheard J, Levy GK, Pitsalidis C, Tan E, Dennis A, Kim JS, Markaki AE, Widera D, Owens RM (2020). Biomimetic and electroactive 3D scaffolds for human neural crest-derived stem cell expansion and osteogenic differentiation. MRS Communications 10: 179–187. https://doi.org/10.1557/mrc.2020.10 [Google Scholar] [CrossRef]
Ingber D (1991). Integrins as mechanochemical transducers. Current Opinion in Cell Biology 3: 841–848. https://doi.org/10.1016/0955-0674(91)90058-7 [Google Scholar] [PubMed] [CrossRef]
Ingber DE (1993). Cellular tensegrity: Defining new rules of biological design that govern the cytoskeleton. Journal of Cell Science 104: 613–627. https://doi.org/10.1242/jcs.104.3.613 [Google Scholar] [PubMed] [CrossRef]
Ingber DE (2006). Cellular mechanotransduction: Putting all the pieces together again. The FASEB Journal 20: 811–827. https://doi.org/10.1096/fj.05-5424rev [Google Scholar] [PubMed] [CrossRef]
Iozzo RV, Schaefer L (2015). Proteoglycan form and function: A comprehensive nomenclature of proteoglycans. Matrix Biology 42: 11–55. https://doi.org/10.1016/j.matbio.2015.02.003 [Google Scholar] [PubMed] [CrossRef]
Jaymand M, Sarvari R, Abbaszadeh P, Massoumi B, Eskandani M, Beygi-Khosrowshahi Y (2016). Development of novel electrically conductive scaffold based on hyperbranched polyester and polythiophene for tissue engineering applications. Journal of Biomedical Materials Research Part A 104: 2673–2684. https://doi.org/10.1002/jbm.a.35811 [Google Scholar] [PubMed] [CrossRef]
Jeong HJ, Nam H, Jang J, Lee SJ (2020). 3D bioprinting strategies for the regeneration of functional tubular tissues and organs. Bioengineering 7: 32. https://doi.org/10.3390/bioengineering7020032 [Google Scholar] [PubMed] [CrossRef]
Jin X, Iwasa S, Okada K, Ooi A, Mitsui K, Mitsumata M (2003). Shear stress-induced collagen XII expression is associated with atherogenesis. Biochemical and Biophysical Research Communications 308: 152–158. https://doi.org/10.1016/S0006-291X(03)01344-5 [Google Scholar] [PubMed] [CrossRef]
Jokhadze G, Machaidze M, Panosyan H, Chu CC, Katsarava R (2007). Synthesis and characterization of functional elastomeric poly(ester amide) co-polymers. Journal of Biomaterials Science, Polymer Edition 18: 411–438. https://doi.org/10.1163/156856207780425031 [Google Scholar] [PubMed] [CrossRef]
Julian L, Olson MF (2014). Rho-associated coiled-coil containing kinases (ROCK) structure, regulation, and functions. Small GTPases 5: e29846. https://doi.org/10.4161/sgtp.29846 [Google Scholar] [PubMed] [CrossRef]
Juliano RL, Haskill S (1993). Signal transduction from the extracellular matrix. The Journal of Cell Biology 120: 577–585. https://doi.org/10.1083/jcb.120.3.577 [Google Scholar] [PubMed] [CrossRef]
Jun I, Han HS, Edwards JR, Jeon H (2018). Electrospun fibrous scaffolds for tissue engineering: Viewpoints on architecture and fabrication. International Journal of Molecular Sciences 19: 745. https://doi.org/10.3390/ijms19030745 [Google Scholar] [PubMed] [CrossRef]
Karakurt I, Lin L (2020). 3D printing technologies: Techniques, materials, and post-processing. Current Opinion in Chemical Engineering 28: 134–143. https://doi.org/10.1016/j.coche.2020.04.001 [Google Scholar] [CrossRef]
Karamichos D, Brown R, Mudera V (2006). Complex dependence of substrate stiffness and serum concentration on cell-force generation. Journal of Biomedical Materials Research Part A 78: 407–415. https://doi.org/10.1002/(ISSN)1552-4965 [Google Scholar] [CrossRef]
Karamichos D, Brown R, Mudera V (2007). Collagen stiffness regulates cellular contraction and matrix remodeling gene expression. Journal of Biomedical Materials Research Part A 83: 887–894. https://doi.org/10.1002/(ISSN)1552-4965 [Google Scholar] [CrossRef]
Karyappa R, Hashimoto M (2019). Chocolate-based ink three-dimensional printing (Ci3DP). Scientific Reports 9: 1–11. https://doi.org/10.1038/s41598-019-50583-5 [Google Scholar] [PubMed] [CrossRef]
Kelly CN, Wang T, Crowley J, Wills D, Pelletier MH, Westrick ER, Adams SB, Gall K, Walsh WR (2021). High-strength, porous additively manufactured implants with optimized mechanical osseointegration. Biomaterials 279: 121206. https://doi.org/10.1016/j.biomaterials.2021.121206 [Google Scholar] [PubMed] [CrossRef]
Khetan S, Guvendiren M, Legant WR, Cohen DM, Chen CS, Burdick JA (2013). Degradation-mediated cellular traction directs stem cell fate in covalently crosslinked three-dimensional hydrogels. Nature Materials 12: 458–465. https://doi.org/10.1038/nmat3586 [Google Scholar] [PubMed] [CrossRef]
Khoshnevisan K, Maleki H, Samadian H, Shahsavari S, Sarrafzadeh MH, Larijani B, Dorkoosh FA, Haghpanah V, Khorramizadeh MR (2018). Cellulose acetate electrospun nanofibers for drug delivery systems: Applications and recent advances. Carbohydrate Polymers 198: 131–141. https://doi.org/10.1016/j.carbpol.2018.06.072 [Google Scholar] [PubMed] [CrossRef]
Kilian KA, Bugarija B, Lahn BT, Mrksich M (2010). Geometric cues for directing the differentiation of mesenchymal stem cells. Proceedings of the National Academy of Sciences of the United States of America 107: 4872–4877. https://doi.org/10.1073/pnas.0903269107 [Google Scholar] [PubMed] [CrossRef]
Kim IG, Hwang MP, Du P, Ko J, Ha CW, Do SH, Park K (2015b). Bioactive cell-derived matrices combined with polymer mesh scaffold for osteogenesis and bone healing. Biomaterials 50: 75–86. https://doi.org/10.1016/j.biomaterials.2015.01.054 [Google Scholar] [PubMed] [CrossRef]
Kim JI, Hwang TI, Lee JC, Park CH, Kim CS (2020). Regulating electrical cue and mechanotransduction in topological gradient structure modulated piezoelectric scaffolds to predict neural cell response. Advanced Functional Materials 30: 1907330. https://doi.org/10.1002/adfm.201907330 [Google Scholar] [CrossRef]
Kim BS, Park KE, Kim MH, You HK, Lee J, Park WH (2015a). Effect of nanofiber content on bone regeneration of silk fibroin/poly (ε-caprolactone) nano/microfibrous composite scaffolds. International Journal of Nanomedicine 10: 485. https://doi.org/10.2147/IJN.S72730 [Google Scholar] [PubMed] [CrossRef]
Kim J, Yang C, Kim EJ, Jang J, Kim SJ, Kang SM, Kim MG, Jung H, Park D, Kim C (2016). Vimentin filaments regulate integrin-ligand interactions by binding to the cytoplasmic tail of integrin β3. Journal of Cell Science 129: 2030–2042. https://doi.org/10.1242/jcs.180315 [Google Scholar] [PubMed] [CrossRef]
Klapholz B, Herbert SL, Wellmann J, Johnson R, Parsons M, Brown NH (2015). Alternative mechanisms for talin to mediate integrin function. Current Biology 25: 847–857. https://doi.org/10.1016/j.cub.2015.01.043 [Google Scholar] [PubMed] [CrossRef]
Klinkenberg M, Fischer S, Kremer T, Hernekamp F, Lehnhardt M, Daigeler A (2013). Comparison of anterolateral thigh, lateral arm, and parascapular free flaps with regard to donor-site morbidity and aesthetic and functional outcomes. Plastic and Reconstructive Surgery 131: 293–302. https://doi.org/10.1097/PRS.0b013e31827786bc [Google Scholar] [PubMed] [CrossRef]
Konta AA, García-Piña M, Serrano DR (2017). Personalised 3D printed medicines: Which techniques and polymers are more successful? Bioengineering 4: 79. https://doi.org/10.3390/bioengineering4040079 [Google Scholar] [PubMed] [CrossRef]
Kwee BJ, Mooney DJ (2017). Biomaterials for skeletal muscle tissue engineering. Current Opinion in Biotechnology 47: 16–22. https://doi.org/10.1016/j.copbio.2017.05.003 [Google Scholar] [PubMed] [CrossRef]
Langhans SA (2018). Three-dimensional in vitro cell culture models in drug discovery and drug repositioning. Frontiers in Pharmacology 9: 6. https://doi.org/10.3389/fphar.2018.00006 [Google Scholar] [PubMed] [CrossRef]
Lee YS, Arinzeh TL (2012). The influence of piezoelectric scaffolds on neural differentiation of human neural stem/progenitor cells. Tissue Engineering Part A 18: 2063–2072. https://doi.org/10.1089/ten.tea.2011.0540 [Google Scholar] [PubMed] [CrossRef]
Lee C, Grodzinsky A, Spector M (2001). The effects of cross-linking of collagen-glycosaminoglycan scaffolds on compressive stiffness, chondrocyte-mediated contraction, proliferation and biosynthesis. Biomaterials 22: 3145–3154. https://doi.org/10.1016/S0142-9612(01)00067-9 [Google Scholar] [PubMed] [CrossRef]
Lee JW, Kang KS, Lee SH, Kim JY, Lee BK, Cho DW (2011). Bone regeneration using a microstereolithography-produced customized poly(propylene fumarate)/diethyl fumarate photopolymer 3D scaffold incorporating BMP-2 loaded PLGA microspheres. Biomaterials 32: 744–752. https://doi.org/10.1016/j.biomaterials.2010.09.035 [Google Scholar] [PubMed] [CrossRef]
Lee M, Vasioukhin V (2008). Cell polarity and cancer-cell and tissue polarity as a non-canonical tumor suppressor. Journal of Cell Science 121: 1141–1150. https://doi.org/10.1242/jcs.016634 [Google Scholar] [PubMed] [CrossRef]
Lei L, Liu Z, Yuan P, Jin R, Wang X, Jiang T, Chen X (2019). Injectable colloidal hydrogel with mesoporous silica nanoparticles for sustained co-release of microRNA-222 and aspirin to achieve innervated bone regeneration in rat mandibular defects. Journal of Materials Chemistry B 7: 2722–2735. https://doi.org/10.1039/C9TB00025A [Google Scholar] [PubMed] [CrossRef]
Lemma ED, Spagnolo B, de Vittorio M, Pisanello F (2019). Studying cell mechanobiology in 3D: The two-photon lithography approach. Trends in Biotechnology 37: 358–372. https://doi.org/10.1016/j.tibtech.2018.09.008 [Google Scholar] [PubMed] [CrossRef]
Levy-Mishali M, Zoldan J, Levenberg S (2009). Effect of scaffold stiffness on myoblast differentiation. Tissue Engineering Part A 15: 935–944. https://doi.org/10.1089/ten.tea.2008.0111 [Google Scholar] [PubMed] [CrossRef]
Lewis JA, Smay JE, Stuecker J, Cesarano J (2006). Direct ink writing of three-dimensional ceramic structures. Journal of the American Ceramic Society 89: 3599–3609. https://doi.org/10.1111/j.1551-2916.2006.01382.x [Google Scholar] [CrossRef]
Li S, Kim M, Hu YL, Jalali S, Schlaepfer DD, Hunter T, Chien S, Shyy JY (1997). Fluid shear stress activation of focal adhesion kinase: Linking to mitogen-activated protein kinases. Journal of Biological Chemistry 272: 30455–30462. https://doi.org/10.1074/jbc.272.48.30455 [Google Scholar] [PubMed] [CrossRef]
Li G, Li S, Zhang L, Chen S, Sun Z, Li S, Zhang L, Yang Y (2019). Construction of biofunctionalized anisotropic hydrogel micropatterns and their effect on Schwann cell behavior in peripheral nerve regeneration. ACS Applied Materials & Interfaces 11: 37397–37410. https://doi.org/10.1021/acsami.9b08510 [Google Scholar] [PubMed] [CrossRef]
Li N, Qiao D, Zhao S, Lin Q, Zhang B, Xie F (2021). 3D printing to innovate biopolymer materials for demanding applications: A review. Materials Today Chemistry 20: 100459. https://doi.org/10.1016/j.mtchem.2021.100459 [Google Scholar] [CrossRef]
Liao JK, Seto M, Noma K (2007). Rho kinase (ROCK) inhibitors. Journal of Cardiovascular Pharmacology 50: 17–24. https://doi.org/10.1097/FJC.0b013e318070d1bd [Google Scholar] [PubMed] [CrossRef]
Liu C, Li Y, Zhang Y, Xu H (2022a). The experimental study of regeneration of annulus fibrosus using decellularized annulus fibrosus matrix/poly(ether carbonate urethane) urea-blended fibrous scaffolds with varying elastic moduli. Journal of Biomedical Materials Research Part A 110: 991–1003. https://doi.org/10.1002/jbm.a.37347 [Google Scholar] [PubMed] [CrossRef]
Liu Y, Yang Q, Wang Y, Lin M, Tong Y et al. (2022b). Metallic scaffold with micron-scale geometrical cues promotes osteogenesis and angiogenesis via the ROCK/Myosin/YAP pathway. ACS Biomaterials Science & Engineering 8: 3498–3514. https://doi.org/10.1021/acsbiomaterials.2c00225 [Google Scholar] [PubMed] [CrossRef]
Lo SH (2006). Focal adhesions: What’s new inside. Developmental Biology 294: 280–291. https://doi.org/10.1016/j.ydbio.2006.03.029 [Google Scholar] [PubMed] [CrossRef]
Lovell LG, Lu H, Elliott JE, Stansbury JW, Bowman CN (2001). The effect of cure rate on the mechanical properties of dental resins. Dental Materials 17: 504–511. https://doi.org/10.1016/S0109-5641(01)00010-0 [Google Scholar] [PubMed] [CrossRef]
MacKenna DA, Dolfi F, Vuori K, Ruoslahti E (1998). Extracellular signal-regulated kinase and c-Jun NH2-terminal kinase activation by mechanical stretch is integrin-dependent and matrix-specific in rat cardiac fibroblasts. The Journal of Clinical Investigation 101: 301–310. https://doi.org/10.1172/JCI1026 [Google Scholar] [PubMed] [CrossRef]
Mandala R, Bannoth AP, Akella S, Rangari VK, Kodali D (2022). A short review on fused deposition modeling 3D printing of bio-based polymer nanocomposites. Journal of Applied Polymer Science 139: 51904. https://doi.org/10.1002/app.51904 [Google Scholar] [CrossRef]
Maniotis AJ, Chen CS, Ingber DE (1997). Demonstration of mechanical connections between integrins, cytoskeletal filaments, and nucleoplasm that stabilize nuclear structure. Proceedings of the National Academy of Sciences of the United States of America 94: 849–854. https://doi.org/10.1073/pnas.94.3.849 [Google Scholar] [PubMed] [CrossRef]
Matveeva EA, Venkova LS, Chernoivanenko IS, Minin AA (2015). Vimentin is involved in regulation of mitochondrial motility and membrane potential by Rac1. Biology Open 4: 1290–1297. https://doi.org/10.1242/bio.011874 [Google Scholar] [PubMed] [CrossRef]
McCullen S, Zhu Y, Bernacki S, Narayan R, Pourdeyhimi B, Gorga R, Loboa E (2009). Electrospun composite poly (L-lactic acid)/tricalcium phosphate scaffolds induce proliferation and osteogenic differentiation of human adipose-derived stem cells. Biomedical Materials 4: 035002. https://doi.org/10.1088/1748-6041/4/3/035002 [Google Scholar] [PubMed] [CrossRef]
Moeendarbary E, Harris AR (2014). Cell mechanics: Principles, practices, and prospects. Wiley Interdisciplinary Reviews: Systems Biology and Medicine 6: 371–388. https://doi.org/10.1002/wsbm.1275 [Google Scholar] [PubMed] [CrossRef]
Mohammadalizadeh Z, Karbasi S, Arasteh S (2020). Physical, mechanical and biological evaluation of poly (3-hydroxybutyrate)-chitosan/MWNTs as a novel electrospun scaffold for cartilage tissue engineering applications. Polymer-Plastics Technology and Materials 59: 417–429. https://doi.org/10.1080/25740881.2019.1647244 [Google Scholar] [CrossRef]
Morrison SJ, Kimble J (2006). Asymmetric and symmetric stem-cell divisions in development and cancer. Nature 441: 1068–1074. https://doi.org/10.1038/nature04956 [Google Scholar] [PubMed] [CrossRef]
Muller JM, Chilian WM, Davis MJ (1997). Integrin signaling transduces shear stress-dependent vasodilation of coronary arterioles. Circulation Research 80: 320–326. https://doi.org/10.1161/01.RES.80.3.320 [Google Scholar] [PubMed] [CrossRef]
Munevar S, Wang YL, Dembo M (2004). Regulation of mechanical interactions between fibroblasts and the substratum by stretch-activated Ca2+ entry. Journal of Cell Science 117: 85–92. https://doi.org/10.1242/jcs.00795 [Google Scholar] [PubMed] [CrossRef]
Mücke N, Kreplak L, Kirmse R, Wedig T, Herrmann H, Aebi U, Langowski J (2004). Assessing the flexibility of intermediate filaments by atomic force microscopy. Journal of Molecular Biology 335: 1241–1250. https://doi.org/10.1016/j.jmb.2003.11.038 [Google Scholar] [PubMed] [CrossRef]
Naqvi S, McNamara L (2020). Stem cell mechanobiology and the role of biomaterials in governing mechanotransduction and matrix production for tissue regeneration. Frontiers in Bioengineering and Biotechnology 8: 597661. https://doi.org/10.3389/fbioe.2020.597661 [Google Scholar] [PubMed] [CrossRef]
Nekrasova OE, Mendez MG, Chernoivanenko IS, Tyurin-Kuzmin PA, Kuczmarski ER, Gelfand VI, Goldman RD, Minin AA (2011). Vimentin intermediate filaments modulate the motility of mitochondria. Molecular Biology of the Cell 22: 2282–2289. https://doi.org/10.1091/mbc.e10-09-0766 [Google Scholar] [PubMed] [CrossRef]
Nikbakht M, Karbasi S, Rezayat SM (2020). Biological evaluation of the effects of hyaluronic acid on poly(3-hydroxybutyrate) based electrospun nanocomposite scaffolds for cartilage tissue engineering application. Materials Technology 35: 141–151. https://doi.org/10.1080/10667857.2019.1659535 [Google Scholar] [CrossRef]
Nirmalanandhan VS, Rao M, Shearn JT, Juncosa-Melvin N, Gooch C, Butler DL (2008). Effect of scaffold material, construct length and mechanical stimulation on the in vitro stiffness of the engineered tendon construct. Journal of Biomechanics 41: 822–828. https://doi.org/10.1016/j.jbiomech.2007.11.009 [Google Scholar] [PubMed] [CrossRef]
Novakova-Marcincinova L, Novak-Marcincin J (2012). Testing of materials for rapid prototyping fused deposition modelling technology. International Journal of Industrial and Manufacturing Engineering 6: 2082–2085. https://doi.org/10.5281/zenodo.1055028 [Google Scholar] [CrossRef]
Page RL, Malcuit C, Vilner L, Vojtic I, Shaw S, Hedblom E, Hu J, Pins GD, Rolle MW, Dominko T (2011). Restoration of skeletal muscle defects with adult human cells delivered on fibrin microthreads. Tissue Engineering Part A 17: 2629–2640. https://doi.org/10.1089/ten.tea.2011.0024 [Google Scholar] [PubMed] [CrossRef]
Pan Z, Duan P, Liu X, Wang H, Cao L, He Y, Dong J, Ding J (2015). Effect of porosities of bilayered porous scaffolds on spontaneous osteochondral repair in cartilage tissue engineering. Regenerative Biomaterials 2: 9–19. https://doi.org/10.1093/rb/rbv001 [Google Scholar] [PubMed] [CrossRef]
Parandoush P, Lin D (2017). A review on additive manufacturing of polymer-fiber composites. Composite Structures 182: 36–53. https://doi.org/10.1016/j.compstruct.2017.08.088 [Google Scholar] [CrossRef]
Park GE, Pattison MA, Park K, Webster TJ (2005). Accelerated chondrocyte functions on NaOH-treated PLGA scaffolds. Biomaterials 26: 3075–3082. https://doi.org/10.1016/j.biomaterials.2004.08.005 [Google Scholar] [PubMed] [CrossRef]
Parrish J, Lim K, Baer K, Hooper G, Woodfield T (2018). A 96-well microplate bioreactor platform supporting individual dual perfusion and high-throughput assessment of simple or biofabricated 3D tissue models. Lab on a Chip 18: 2757–2775. https://doi.org/10.1039/C8LC00485D [Google Scholar] [PubMed] [CrossRef]
Patel A, Mukundan S, Wang W, Karumuri A, Sant V, Mukhopadhyay SM, Sant S (2016). Carbon-based hierarchical scaffolds for myoblast differentiation: Synergy between nano-functionalization and alignment. Acta Biomaterialia 32: 77–88. https://doi.org/10.1016/j.actbio.2016.01.004 [Google Scholar] [PubMed] [CrossRef]
Patten J, Wang K (2021). Fibronectin in development and wound healing. Advanced Drug Delivery Reviews 170: 353–368. https://doi.org/10.1016/j.addr.2020.09.005 [Google Scholar] [PubMed] [CrossRef]
Peng B, Chen Y, Leong KW (2015). MicroRNA delivery for regenerative medicine. Advanced Drug Delivery Reviews 88: 108–122. https://doi.org/10.1016/j.addr.2015.05.014 [Google Scholar] [PubMed] [CrossRef]
Pereira D, Richert A, Medjkane S, Hénon S, Weitzman JB (2020). Cell geometry and the cytoskeleton impact the nucleo-cytoplasmic localisation of the SMYD3 methyltransferase. Scientific Reports 10: 1–12. https://doi.org/10.1038/s41598-020-75833-9 [Google Scholar] [PubMed] [CrossRef]
Persson M, Lehenkari PP, Berglin L, Turunen S, Finnilä MA, Risteli J, Skrifvars M, Tuukkanen J (2018). Osteogenic differentiation of human mesenchymal stem cells in a 3D woven scaffold. Scientific Reports 8: 1–12. https://doi.org/10.1038/s41598-018-28699-x [Google Scholar] [PubMed] [CrossRef]
Placone JK, Engler AJ (2018). Recent advances in extrusion-based 3D printing for biomedical applications. Advanced Healthcare Materials 7: 1701161. https://doi.org/10.1002/adhm.201701161 [Google Scholar] [PubMed] [CrossRef]
Plow EF, Qin J (2019). The kindlin family of adapter proteins: A past, present, and future prospectus. Circulation Research 124: 202–204. https://doi.org/10.1161/CIRCRESAHA.118.314362 [Google Scholar] [PubMed] [CrossRef]
Potard U, Butler JP, Wang N (1997). Cytoskeletal mechanics in confluent epithelial cells probed through integrins and E-cadherins. American Journal of Physiology-Cell Physiology 272: C1654–C1663. https://doi.org/10.1152/ajpcell.1997.272.5.C1654 [Google Scholar] [PubMed] [CrossRef]
Potts JR, Campbell ID (1994). Fibronectin structure and assembly. Current Opinion in Cell Biology 6: 648–655. https://doi.org/10.1016/0955-0674(94)90090-6 [Google Scholar] [PubMed] [CrossRef]
Puklin-Faucher E, Sheetz MP (2009). The mechanical integrin cycle. Journal of Cell Science 122: 179–186. https://doi.org/10.1242/jcs.042127 [Google Scholar] [PubMed] [CrossRef]
Qin L, Fu X, Ma J, Lin M, Zhang P, Wang Y, Yan Q, Tao C, Liu W, Tang B (2021). Kindlin-2 mediates mechanotransduction in bone by regulating expression of Sclerostin in osteocytes. Communications Biology 4: 1–15. https://doi.org/10.1038/s42003-021-01950-4 [Google Scholar] [PubMed] [CrossRef]
Reinhart-King CA, Dembo M, Hammer DA (2008). Cell-cell mechanical communication through compliant substrates. Biophysical Journal 95: 6044–6051. https://doi.org/10.1529/biophysj.107.127662 [Google Scholar] [PubMed] [CrossRef]
Romani P, Valcarcel-Jimenez L, Frezza C, Dupont S (2021). Crosstalk between mechanotransduction and metabolism. Nature Reviews Molecular Cell Biology 22: 22–38. https://doi.org/10.1038/s41580-020-00306-w [Google Scholar] [PubMed] [CrossRef]
Rose JC, Cámara-Torres M, Rahimi K, Köhler J, Möller M, de Laporte L (2017). Nerve cells decide to orient inside an injectable hydrogel with minimal structural guidance. Nano Letters 17: 3782–3791. https://doi.org/10.1021/acs.nanolett.7b01123 [Google Scholar] [PubMed] [CrossRef]
Roseti L, Parisi V, Petretta M, Cavallo C, Desando G, Bartolotti I, Grigolo B (2017). Scaffolds for bone tissue engineering: State of the art and new perspectives. Materials Science and Engineering C 78: 1246–1262. https://doi.org/10.1016/j.msec.2017.05.017 [Google Scholar] [PubMed] [CrossRef]
Ruiz SA, Chen CS (2007). Microcontact printing: A tool to pattern. Soft Matter 3: 168–177. https://doi.org/10.1039/B613349E [Google Scholar] [PubMed] [CrossRef]
Saadi M, Maguire A, Pottackal NT, Thakur MSH, Ikram MM, Hart AJ, Ajayan PM, Rahman MM (2022). Direct ink writing: A 3D printing technology for diverse materials. Advanced Materials 34: 2108855. https://doi.org/10.1002/adma.202108855 [Google Scholar] [PubMed] [CrossRef]
Salter D, Robb J, Wright M (1997). Electrophysiological responses of human bone cells to mechanical stimulation: Evidence for specific integrin function in mechanotransduction. Journal of Bone and Mineral Research 12: 1133–1141. https://doi.org/10.1359/jbmr.1997.12.7.1133 [Google Scholar] [PubMed] [CrossRef]
Schenke-Layland K, Nsair A, Van Handel B, Angelis E, Gluck JM, Votteler M, Goldhaber JI, Mikkola HK, Kahn M, MacLellan WR (2011). Recapitulation of the embryonic cardiovascular progenitor cell niche. Biomaterials 32: 2748–2756. https://doi.org/10.1016/j.biomaterials.2010.12.046 [Google Scholar] [PubMed] [CrossRef]
Schoenwaelder SM, Burridge K (1999). Bidirectional signaling between the cytoskeleton and integrins. Current Opinion in Cell Biology 11: 274–286. https://doi.org/10.1016/S0955-0674(99)80037-4 [Google Scholar] [PubMed] [CrossRef]
Schwander M, Kachar B, Müller U (2010). Review series: The cell biology of hearing. The Journal of Cell Biology 190: 9–20. https://doi.org/10.1083/jcb.201001138 [Google Scholar] [PubMed] [CrossRef]
Sears NA, Seshadri DR, Dhavalikar PS, Cosgriff-Hernandez E (2016). A review of three-dimensional printing in tissue engineering. Tissue Engineering Part B: Reviews 22: 298–310. https://doi.org/10.1089/ten.teb.2015.0464 [Google Scholar] [PubMed] [CrossRef]
Seidlits SK, Liang J, Bierman RD, Sohrabi A, Karam J, Holley SM, Cepeda C, Walthers CM (2019). Peptide-modified, hyaluronic acid-based hydrogels as a 3D culture platform for neural stem/progenitor cell engineering. Journal of Biomedical Materials Research Part A 107: 704–718. https://doi.org/10.1002/jbm.a.36603 [Google Scholar] [PubMed] [CrossRef]
Septiadi D, Crippa F, Moore TL, Rothen-Rutishauser B, Petri-Fink A (2018). Nanoparticle-cell interaction: A cell mechanics perspective. Advanced Materials 30: 1704463. https://doi.org/10.1002/adma.201704463 [Google Scholar] [PubMed] [CrossRef]
Seyedjafari E, Soleimani M, Ghaemi N, Shabani I (2010). Nanohydroxyapatite-coated electrospun poly(l-lactide) nanofibers enhance osteogenic differentiation of stem cells and induce ectopic bone formation. Biomacromolecules 11: 3118–3125. https://doi.org/10.1021/bm1009238 [Google Scholar] [PubMed] [CrossRef]
Shanmugam V, Pavan MV, Babu K, Karnan B (2021). Fused deposition modeling based polymeric materials and their performance: A review. Polymer Composites 42: 5656–5677. https://doi.org/10.1002/pc.26275 [Google Scholar] [CrossRef]
Shyy JY-J, Chien S (2002). Role of integrins in endothelial mechanosensing of shear stress. Circulation Research 91: 769–775. https://doi.org/10.1161/01.RES.0000038487.19924.18 [Google Scholar] [PubMed] [CrossRef]
Silva GA, Czeisler C, Niece KL, Beniash E, Harrington DA, Kessler JA, Stupp SI (2004). Selective differentiation of neural progenitor cells by high-epitope density nanofibers. Science 303: 1352–1355. https://doi.org/10.1126/science.1093783 [Google Scholar] [PubMed] [CrossRef]
Spiegel CA, Hippler M, Münchinger A, Bastmeyer M, Barner-Kowollik C, Wegener M, Blasco E (2020). 4D printing at the microscale. Advanced Functional Materials 30: 1907615. https://doi.org/10.1002/adfm.201907615 [Google Scholar] [CrossRef]
Sun W, Incitti T, Migliaresi C, Quattrone A, Casarosa S, Motta A (2017). Viability and neuronal differentiation of neural stem cells encapsulated in silk fibroin hydrogel functionalized with an IKVAV peptide. Journal of Tissue Engineering and Regenerative Medicine 11: 1532–1541. https://doi.org/10.1002/term.2053 [Google Scholar] [PubMed] [CrossRef]
Tenje M, Cantoni F, Hernández AMP, Searle SS, Johansson S, Barbe L, Antfolk M, Pohlit H (2020). A practical guide to microfabrication and patterning of hydrogels for biomimetic cell culture scaffolds. Organs-on-a-Chip 2: 100003. https://doi.org/10.1016/j.ooc.2020.100003 [Google Scholar] [CrossRef]
Theocharis AD, Manou D, Karamanos NK (2019). The extracellular matrix as a multitasking player in disease. The FEBS Journal 286: 2830–2869. https://doi.org/10.1111/febs.14818 [Google Scholar] [PubMed] [CrossRef]
Tomaskovic-Crook E, Gu Q, Rahim SNA, Wallace GG, Crook JM (2020). Conducting polymer mediated electrical stimulation induces multilineage differentiation with robust neuronal fate determination of human induced pluripotent stem cells. Cells 9: 658. https://doi.org/10.3390/cells9030658 [Google Scholar] [PubMed] [CrossRef]
Tomaskovic-Crook E, Zhang P, Ahtiainen A, Kaisvuo H, Lee CY, Beirne S, Aqrawe Z, Svirskis D, Hyttinen J, Wallace GG (2019). Human neural tissues from neural stem cells using conductive biogel and printed polymer microelectrode arrays for 3D electrical stimulation. Advanced Healthcare Materials 8: 1900425. https://doi.org/10.1002/adhm.201900425 [Google Scholar] [PubMed] [CrossRef]
Varma MV, Kandasubramanian B, Ibrahim SM (2020). 3D printed scaffolds for biomedical applications. Materials Chemistry and Physics 255: 123642. https://doi.org/10.1016/j.matchemphys.2020.123642 [Google Scholar] [CrossRef]
Ventre M, Coppola V, Natale CF, Netti PA (2019). Aligned fibrous decellularized cell derived matrices for mesenchymal stem cell amplification. Journal of Biomedical Materials Research Part A 107: 2536–2546. https://doi.org/10.1002/jbm.a.36759 [Google Scholar] [PubMed] [CrossRef]
Vining KH, Mooney DJ (2017). Mechanical forces direct stem cell behaviour in development and regeneration. Nature Reviews Molecular Cell Biology 18: 728–742. https://doi.org/10.1038/nrm.2017.108 [Google Scholar] [PubMed] [CrossRef]
Vyavahare S, Teraiya S, Panghal D, Kumar S (2019). Fused deposition modelling: A review. Rapid Prototyping Journal 26: 176–201. https://doi.org/10.1108/RPJ-04-2019-0106 [Google Scholar] [CrossRef]
Wan S, Fu X, Ji Y, Li M, Shi X, Wang Y (2018). FAK- and YAP/TAZ dependent mechanotransduction pathways are required for enhanced immunomodulatory properties of adipose-derived mesenchymal stem cells induced by aligned fibrous scaffolds. Biomaterials 171: 107–117. https://doi.org/10.1016/j.biomaterials.2018.04.035 [Google Scholar] [PubMed] [CrossRef]
Wan X, Luo L, Liu Y, Leng J (2020). Direct ink writing based 4D printing of materials and their applications. Advanced Science 7: 2001000. https://doi.org/10.1002/advs.202001000 [Google Scholar] [PubMed] [CrossRef]
Wang N, Butler JP, Ingber DE (1993). Mechanotransduction across the cell surface and through the cytoskeleton. Science 260: 1124–1127. https://doi.org/10.1126/science.7684161 [Google Scholar] [PubMed] [CrossRef]
Wang S, Guan S, Li W, Ge D, Xu J, Sun C, Liu T, Ma X (2018a). 3D culture of neural stem cells within conductive PEDOT layer-assembled chitosan/gelatin scaffolds for neural tissue engineering. Materials Science and Engineering C 93: 890–901. https://doi.org/10.1016/j.msec.2018.08.054 [Google Scholar] [PubMed] [CrossRef]
Wang N, Ingber DE (1995). Probing transmembrane mechanical coupling and cytomechanics using magnetic twisting cytometry. Biochemistry and Cell Biology 73: 327–335. https://doi.org/10.1139/o95-041 [Google Scholar] [PubMed] [CrossRef]
Wang H, Liu Y, Chen Z, Sun L, Zhao Y (2020a). Anisotropic structural color particles from colloidal phase separation. Science Advances 6: eaay1438. https://doi.org/10.1126/sciadv.aay1438 [Google Scholar] [PubMed] [CrossRef]
Wang WY, Pearson AT, Kutys ML, Choi CK, Wozniak MA, Baker BM, Chen CS (2018b). Extracellular matrix alignment dictates the organization of focal adhesions and directs uniaxial cell migration. APL Bioengineering 2: 046107. https://doi.org/10.1063/1.5052239 [Google Scholar] [PubMed] [CrossRef]
Wang N, Planus E, Pouchelet M, Fredberg JJ, Barlovatz-Meimon G (1995). Urokinase receptor mediates mechanical force transfer across the cell surface. American Journal of Physiology-Cell Physiology 268: C1062–C1066. https://doi.org/10.1152/ajpcell.1995.268.4.C1062 [Google Scholar] [PubMed] [CrossRef]
Wang JHC, Thampatty BP (2008). Mechanobiology of adult and stem cells. International Review of Cell and Molecular Biology 271: 301–346. https://doi.org/10.1016/S1937-6448(08)01207-0 [Google Scholar] [PubMed] [CrossRef]
Wang X, Wang G, Zingales S, Zhao B (2018c). Biomaterials enabled cell-free strategies for endogenous bone regeneration. Tissue Engineering Part B: Reviews 24: 463–481. https://doi.org/10.1089/ten.teb.2018.0012 [Google Scholar] [PubMed] [CrossRef]
Wang L, Wu Y, Hu T, Ma PX, Guo B (2019). Aligned conductive core-shell biomimetic scaffolds based on nanofiber yarns/hydrogel for enhanced 3D neurite outgrowth alignment and elongation. Acta Biomaterialia 96: 175–187. https://doi.org/10.1016/j.actbio.2019.06.035 [Google Scholar] [PubMed] [CrossRef]
Wang X, Wu D, Li W, Yang L (2021). Emerging biomaterials for reproductive medicine. Engineered Regeneration 2: 230–245. https://doi.org/10.1016/j.engreg.2021.11.006 [Google Scholar] [CrossRef]
Wang J, Xiong H, Zhu T, Liu Y, Pan H, Fan C, Zhao X, Lu WW (2020b). Bioinspired multichannel nerve guidance conduit based on shape memory nanofibers for potential application in peripheral nerve repair. ACS Nano 14: 12579–12595. https://doi.org/10.1021/acsnano.0c03570 [Google Scholar] [PubMed] [CrossRef]
Watters MP, Bernhardt ML (2018). Curing parameters to improve the mechanical properties of stereolithographic printed specimens. Rapid Prototyping Journal 24: 46–51. https://doi.org/10.1108/RPJ-11-2016-0180 [Google Scholar] [CrossRef]
Wei Y, Lukashev M, Simon DI, Bodary SC, Rosenberg S, Doyle MV, Chapman HA (1996). Regulation of integrin function by the urokinase receptor. Science 273: 1551–1555. https://doi.org/10.1126/science.273.5281.1551 [Google Scholar] [PubMed] [CrossRef]
Weißenbruch K, Lemma ED, Hippler M, Bastmeyer M (2022). Micro-scaffolds as synthetic cell niches: Recent advances and challenges. Current Opinion in Biotechnology 73: 290–299. https://doi.org/10.1016/j.copbio.2021.08.016 [Google Scholar] [PubMed] [CrossRef]
Wiche G (1998). Role of plectin in cytoskeleton organization and dynamics. Journal of Cell Science 111: 2477–2486. https://doi.org/10.1242/jcs.111.17.2477 [Google Scholar] [PubMed] [CrossRef]
Wilson E, Sudhir K, Ives HE (1995). Mechanical strain of rat vascular smooth muscle cells is sensed by specific extracellular matrix/integrin interactions. The Journal of Clinical Investigation 96: 2364–2372. https://doi.org/10.1172/JCI118293 [Google Scholar] [PubMed] [CrossRef]
Wodarz A, Näthke I (2007). Cell polarity in development and cancer. Nature Cell Biology 9: 1016–1024. https://doi.org/10.1038/ncb433 [Google Scholar] [PubMed] [CrossRef]
Wu C, Liu A, Chen S, Zhang X, Chen L, Zhu Y, Xiao Z, Sun J, Luo H, Fan H (2019). Cell-laden electroconductive hydrogel simulating nerve matrix to deliver electrical cues and promote neurogenesis. ACS Applied Materials & Interfaces 11: 22152–22163. https://doi.org/10.1021/acsami.9b05520 [Google Scholar] [PubMed] [CrossRef]
Wu S, Xu R, Duan B, Jiang P (2017). Three-dimensional hyaluronic acid hydrogel-based models for in vitro human iPSC-derived NPC culture and differentiation. Journal of Materials Chemistry B 5: 3870–3878. https://doi.org/10.1039/C7TB00721C [Google Scholar] [PubMed] [CrossRef]
Xie J, Peng C, Zhao Q, Wang X, Yuan H, Yang L, Li K, Lou X, Zhang Y (2016). Osteogenic differentiation and bone regeneration of iPSC-MSCs supported by a biomimetic nanofibrous scaffold. Acta Biomaterialia 29: 365–379. https://doi.org/10.1016/j.actbio.2015.10.007 [Google Scholar] [PubMed] [CrossRef]
Xu J, Xie Y, Zhang H, Ye Z, Zhang W (2014). Fabrication of PLGA/MWNTs composite electrospun fibrous scaffolds for improved myogenic differentiation of C2C12 cells. Colloids and Surfaces B: Biointerfaces 123: 907–915. https://doi.org/10.1016/j.colsurfb.2014.10.041 [Google Scholar] [PubMed] [CrossRef]
Yan J, Yao M, Goult BT, Sheetz MP (2015). Talin dependent mechanosensitivity of cell focal adhesions. Cellular and Molecular Bioengineering 8: 151–159. https://doi.org/10.1007/s12195-014-0364-5 [Google Scholar] [PubMed] [CrossRef]
Yankov E, Nikolova MP (2017). Comparison of the accuracy of 3D printed prototypes using the stereolithography (SLA) method with the digital CAD models. MATEC Web of Conferences (EDP Sciences)Bulgaria. [Google Scholar]
Yao L, Billiar KL, Windebank AJ, Pandit A (2010). Multichanneled collagen conduits for peripheral nerve regeneration: Design, fabrication, and characterization. Tissue Engineering Part C: Methods 16: 1585–1596. https://doi.org/10.1089/ten.tec.2010.0152 [Google Scholar] [PubMed] [CrossRef]
Yao S, Yu S, Cao Z, Yang Y, Yu X, Mao HQ, Wang LN, Sun X, Zhao L, Wang X (2018). Hierarchically aligned fibrin nanofiber hydrogel accelerated axonal regrowth and locomotor function recovery in rat spinal cord injury. International Journal of Nanomedicine 13: 2883. https://doi.org/10.2147/IJN.S159356 [Google Scholar] [PubMed] [CrossRef]
Yoshida M, Westlin WF, Wang N, Ingber DE, Rosenzweig A, Resnick N, GimbroneJr MA (1996). Leukocyte adhesion to vascular endothelium induces E-selectin linkage to the actin cytoskeleton. The Journal of Cell Biology 133: 445–455. https://doi.org/10.1083/jcb.133.2.445 [Google Scholar] [PubMed] [CrossRef]
Yu T, Wen L, He J, Xu Y, Li T et al. (2020). Fabrication and evaluation of an optimized acellular nerve allograft with multiple axial channels. Acta Biomaterialia 115: 235–249. https://doi.org/10.1016/j.actbio.2020.07.059 [Google Scholar] [PubMed] [CrossRef]
Zhang ZC, Li Pl, Chu Ft, Shen G (2019). Influence of the three-dimensional printing technique and printing layer thickness on model accuracy. Journal of Orofacial Orthopedics/Fortschritte der Kieferorthopädie 80: 194–204. https://doi.org/10.1007/s00056-019-00180-y [Google Scholar] [PubMed] [CrossRef]
Zhang R, Ma PX (2000). Synthetic nano-fibrillar extracellular matrices with predesigned macroporous architectures. Journal of Biomedical Materials Research 52: 430–438. https://doi.org/10.1002/(ISSN)1097-4636 [Google Scholar] [CrossRef]
Zhang H, Zhang H, Wang H, Zhao Y, Chai R (2022). Natural proteins-derived asymmetric porous conduit for peripheral nerve regeneration. Applied Materials Today 27: 101431. https://doi.org/10.1016/j.apmt.2022.101431 [Google Scholar] [CrossRef]
Zheng C, Yang Z, Chen S, Zhang F, Rao Z, Zhao C, Quan D, Bai Y, Shen J (2021). Nanofibrous nerve guidance conduits decorated with decellularized matrix hydrogel facilitate peripheral nerve injury repair. Theranostics 11: 2917–2931. https://doi.org/10.7150/thno.50825 [Google Scholar] [PubMed] [CrossRef]
Zhu M, Li W, Dong X, Yuan X, Midgley AC, Chang H, Wang Y, Wang H, Wang K, Ma PX (2019). In vivo engineered extracellular matrix scaffolds with instructive niches for oriented tissue regeneration. Nature Communications 10: 1–14. https://doi.org/10.1038/s41467-019-12545-3 [Google Scholar] [PubMed] [CrossRef]
Zhu C, Li J, Liu C, Zhou P, Yang H, Li B (2016). Modulation of the gene expression of annulus fibrosus-derived stem cells using poly(ether carbonate urethane)urea scaffolds of tunable elasticity. Acta Biomaterialia 29: 228–238. https://doi.org/10.1016/j.actbio.2015.09.039 [Google Scholar] [PubMed] [CrossRef]
Cite This Article
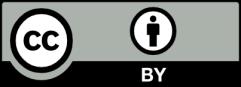
This work is licensed under a Creative Commons Attribution 4.0 International License , which permits unrestricted use, distribution, and reproduction in any medium, provided the original work is properly cited.