Open Access
REVIEW
ROS-hormone interaction in regulating integrative défense signaling of plant cell
1 Plant Physiology and Biochemistry Research Laboratory, Department of Botany, University of Burdwan, Burdwan, 713104, India
2 Department of Botany, University of Rajsahi, Rajsahi, 6205, Bangladesh
* Corresponding Author: SOUMEN BHATTACHARJEE. Email:
(This article belongs to the Special Issue: Physiology and Molecular Biology of Plant Stress Tolerance)
BIOCELL 2023, 47(3), 503-521. https://doi.org/10.32604/biocell.2023.025744
Received 31 July 2022; Accepted 24 October 2022; Issue published 03 January 2023
Abstract
The elaborate redox network of the cell, comprising of events like turnover of reactive oxygen species (ROS), redox sensing, signaling, expression of redox-sensitive genes, etc., often orchestrates with other bonafide hormonal signaling pathways through their synergistic or antagonistic action in the plant cell. The redox cue generated in plant cells under fluctuating environmental conditions can significantly influence other hormonal biosynthetic or signaling mechanisms, thereby modulating physiology towards stress acclimation and defense. There is also strong evidence of the recruitment of ROS as a ‘second messenger’ in different hormonal signaling pathways under stress. Moreover, the retrograde signaling initiated by ROS also found to strongly influence hormonal homeostasis and signaling. The present review, in this aspect, is an effort towards understanding the regulatory roles of ROS in integrating and orchestrating other hormonal signaling pathways or vice versa so as to unfold the relationship between these two signaling episodes of plant cells under environmental odds. We also accentuate the significance of understanding the utterly complex interactions, which occur both at metabolic and genetic levels between ROS and phytohormones during stress combinations. Furthermore, the significant and decisive role of ROS turnover, particularly the contribution of RBOH (respiratory burst oxidase homologs) in the synergism of redox and hormone signaling during systemic acquired acclimation under stress is also discussed.Keywords
Being sessile and poikilothermic, plants are often exposed to several environmental stresses, individually or in combination. These environmental inputs, mostly non-conducive, need to be processed systematically for appropriate adaptive response in plant cells for their survival. Plant growth regulators or hormones could be the prime agents in orchestrating and processing the odd environmental cues necessary for conferring stress acclimation (Suzuki et al., 2016; Souri et al., 2020; Skalak et al., 2021). Therefore, specific regulation of synthesis and functional control of growth regulators are prerequisites that determine plant performance under stresses. Hormones, particularly abscisic acid (ABA), salicylic acid (SA), ethylene (C2H4), jasmonic acid (JA), gibberellic acid (GA), indole-acetic acid (IAA), are known to regulate plant perception of unfavorable environmental cues and transduce to an adaptive response. The roles of these hormones in integrating environmental stress with adaptive response and morphogenesis have been known for a long time (Verma et al., 2016; Souri et al., 2020; Skalak et al., 2021). The last three decades also witnessed several works that strongly convey the significance of redox biology in regulating the stress tolerance of plants (Anjum et al., 2016; Mittler, 2017; Bhattacharjee, 2019; Sies, 2021). In fact, in plants, apart from stress sensing, reactive oxygen species (ROS) control different environmental cues and regulate the initiation of different stress-response networks, thus significantly contributing to the defense mechanisms toward climate resilience (Bhattacharjee, 2019; Kollist et al., 2019; Mittler et al., 2022; Martin et al., 2022). Works in the domain of plant redox biology strongly advocated the position of ROS as signaling molecule with several selective advantages, like their ability to have dynamic changes in their endogenous titer, their control over sub-cellular localization, the ability of long-distance transfer of cell propagating signals and above all, their linkage with cellular metabolism (Fichman and Mittler, 2020; Mittler et al., 2011; Mittler, 2017; Bhattacharjee, 2019). The literature survey also proposes a strong relationship between ROS with other hormonal signaling for integrating responses under different environmental inputs. In fact, many works suggest the role of redox signaling in regulating the functioning of other hormones for processing information of unfavorable environmental cues necessary for appropriate response (Mittler et al., 2011, 2022; Bhattacharjee, 2012; Bartoli et al., 2013; Foyer and Noctor, 2013). Several works in this aspect proposed the feed-forward and backward interaction between hormonal and ROS signaling, necessary for the perception of unfavorable environmental cues, stress acclimation, and developmental processes (Table 1, Fig. 1). The stress acclimation performances, like regulation of stomatal conductance, hydraulic conductivity, secondary root formation, etc., which were previously thought to be regulated by hormonal signaling, now proved to be largely influenced and regulated by ROS-hormone interaction (Sakamoto et al., 2008; Miller et al., 2010; Bhattacharjee, 2019).
Figure 1: Diagram showing impact of environmental stress on hormonal and cellular redox homeostasis that leads to stress acclimatory and apoptotic pathways.
There is also evidence of both upstream and downstream redox regulation of hormonal pathways, where ROS not only act as a secondary messenger in downstream pathways of hormone signaling but can also influence hormone signaling by interfering with their biosynthesis and signaling episodes (Chen et al., 1993; Mittler, 2017). For example, hydrogen peroxide (H2O2) has been found to be recruited as a secondary messenger during ABA signaling and is also found to be involved in the up-regulation of the synthesis of ABA, C2H4 and SA (León et al., 1995). Further, plant growth regulators or phytohormones like auxin, GAs, cytokinin (CKs), ABA, ethylene, SA, JA, brassinosteroids (BR), and strigolactones (SLs) may initiate important signaling cascades for stress adaptation. These signaling cascades do not act in isolation; rather, these pathways are interfered with and regulated by ROS, which can subsequently change the course of the signaling pathway in response to environmental stimuli (Peleg and Blumwald, 2011). With evolution, plants have developed several redox-sensitive proteins, some of which are the components of hormone signaling, that can modulate metabolism in response to stress. Those redox-sensitive molecules are mostly transcription factors (TFs) or protein molecules that act on several other TFs directly or indirectly, which can subsequently change an array of gene expression (Bhattacharjee, 2012; Sharma et al., 2012; Mittler et al., 2022). Most stress hormones (ABA, ethylene, SA, etc.) are linked to the redox status of a cell or directly to the endogenous titer of ROS for their functioning and controlling stress response mechanisms. According to Mohanta et al. (2018), divalent calcium (Ca2+) and ROS are the most versatile signaling molecules involved in the early events of abiotic stress signaling. Ca2+ ion outburst due to a stress response activates downstream calcium and ROS signaling through activation of several signaling intermediates and molecules, including Ca2+-dependent protein kinases (CPK), calmodulin (CaM), calmodulin-like-proteins (CML), calcineurin B-like (CBL), and NADPH-oxidase, which further leads to regulation of the mitogen-activated protein kinases (MAPK) pathway and hormonal signaling cascades. Further, these signaling cascades activate downstream ABA, GA, BR, and nitric oxide (NO) systems and maintain cellular homeostasis. Activated cytosolic CPK, CaM, CML, and CBL further regulates NADPH-oxidase and mediates ROS production in a regulatory feedback mechanism, suggesting that early signaling events in plant abiotic stress responses are regulated through feedback mechanisms. Accordingly, a strong linkage showing feed forward and backward interaction between redox and hormone signaling towards the perception of environmental cues regulating plant performance under stress (Fig. 1) is quite evident from previous studies. Since the perception of unfavorable environmental cues and subsequent signal transduction to appropriate mechanisms towards acclamatory stress tolerance is one of the most important avenues in plant stress biology, understanding the intricate association between the bona fide hormonal signaling pathway with redox signaling deserves special attention. The present review is, therefore, an endeavor toward understanding the cellular language associated with integrative hormonal and redox signaling that regulates systemic responses of plants under environmental stresses. Furthermore, the origin of biological signals in the form of redox cues under environmental stresses and their combinations with hormonal signals is also a matter of great interest in view of the maintenance of the health of the plant under stress and hence also, the subject of discussion (Mittler et al., 2022; Martin et al., 2022).
Integrative redox and hormone signaling under stress combination in plant cell
Though most of the studies related to plant responses to environmental stress centered around the solitary stress effect, in the field condition, plants are often encountered multiple stresses (Mittler, 2006, 2017). Under in vivo system, to acclimatize stress combinations, different stress-responsive signaling mechanisms need to be integrated where the signaling intermediates cross-talk (Mittler, 2006; Choudhury et al., 2017). Further, climate change has introduced unpredictable imposition of stress combinations, which attracted the attention of stress biologists in recent times (Zandalinas et al., 2018, 2020a; Devireddy et al., 2021). Instead of having an additive effect, stress combinations imposed by climate change have been found to have unique responses, which have been substantiated in some recent works (Choudhury et al., 2017; Zandalinas et al., 2018, 2020a; Devireddy et al., 2021). Since most of the system-level responses of plants under environmental stresses are regulated by signaling episodes of plant growth regulators (PGRs) or hormones, their interactions under stress combinations are inevitable to settle the mutual interest of plants. The hormones ABA and ethylene, as well as their combination, have been proposed as key regulators of stress responses (Suzuki et al., 2016; Devireddy et al., 2021). There are many examples of ABA-induced transcriptional reprogramming of stress-responsive genes under multiple stresses like salinity, drought, and hyperthermia (Suzuki et al., 2016). Similarly, drought, and hyperthermia stress combinations subjected to some crops are also responsible for de novo gene expression necessary for survival (Zandalinas et al., 2016, 2018; Balfagόn et al., 2019). Though much emphasis has been given to the functional role of ABA under drought and salinity, the complex coordination with other hormone-signaling pathways cannot be ruled out. The high level of SA, ethylene, and JA are also associated with hyperthermia and salinity stress, highlighting their integrative action in regulating acclamatory response under stress combinations (Balfagόn et al., 2019; Devireddy et al., 2021).
While the hormonal signaling processes ensue under stress combinations, the role of redox players cannot be ruled out, as revealed by several recent studies (Choudhury et al., 2017; Mittler, 2017; Devireddy et al., 2021). Different combination of multiple stresses like extreme temperature, drought, salinity, and excess photochemical energy (EPE) always results in different ROS signature under hormonal signaling, governing specificity of stress acclamatory response (Choudhury et al., 2017; Devireddy et al., 2021). The work of several other workers in the recent past (Choudhury et al., 2017; Mittler, 2017; Zandalinas et al., 2018) also corroborated strongly with the role of redox cues under stress combinations. The endogenous level of pro-oxidants, involvement of antioxidative defense system (ascorbate-glutathione cycle, flavonols, polyphenolic compounds, carotenoid and tocopherol, anthocyanin, betacyanin, and alkaloids), accumulation of redox osmolytes exhibit unique response pattern under stress combinations (Choudhury et al., 2017; Devireddy et al., 2021). In the backdrop of the intricate interplay of different phytohormones along with the involvement of ROS as the second messenger, the complexity of ROS-hormone interaction under multiple stress combinations is inherent. In order to make these signaling co–ordinations successful in regulating plant survival under stress combination, the combinatorial interaction must be flexible depending on stress types and their impact on specific plants (Suzuki et al., 2016; Devireddy et al., 2021). For example, the interaction between ABA, JA, SA, and ROS is required for the regulation of stomatal conductance under stress combinations. Previous studies based on genetic and molecular experiments reported that ABA-sensitive mutant (abi1), with impaired ABA and associated protein ABI1, become sensitive to stress combinations of hyperthermia and drought as well as hyperthermia and salinity (Zandalinas et al., 2016; Suzuki et al., 2016), substantiating the significance of ABA–ROS interaction for stress combinations. Independent studies have also shown that the hormones SA and JA also interact with H2O2 under the same stress combinations for influencing stomatal regulations. Further, the role of other hormone signaling is highlighted in influencing redox signature for stomatal regulation. Other studies of ROS–hormone interaction under stress combinations also revealed the role of JA and CK in controlling redox cues under stress combinations. Although JA up-regulates the expression of the APX1 (ascorbate peroxidase 1) gene under the stress combinations of heat and drought, the impact seems to be on the accumulation of CK as well, which exhibits down-regulation (through the expression of CK degrading enzymes) (Lubovská et al., 2014). Different recent works related to different integrative systemic signaling of ROS and plant growth under stress combinations revealed that the plant could simultaneously process and integrate different systemic signals under stress combinations depending on how efficiently they can relay the ROS signal and integrate the same with hormonal responses (Zandalinas et al., 2016; Devireddy et al., 2021). Exposure of plant leaves to hyperthermia and excess photochemical energy separately triggers the origin of efficient ROS signal that culminates in systemic acquired resistance to both hyperthermia and excess photochemical energy (EPE). On the contrary, imposition of both EPE and hyperthermia simultaneously to the leaves of the same plants inhibited the initiation of ROS waves or redox signals, thereby impairing systemic acquired acclimation under these stress combinations. Incidentally, this suppression of the origin of systemic acquired acclimation was found to be erased in JA aos mutant, strongly vouching for the synergistic interactions between JA and SA in determining systemic signaling under stress combinations (Zandalinas et al., 2020b) through the specific ROS–hormone interactions. All such experimental evidence (Table 1) strongly corroborates the specific integration pattern between ROS–hormone signaling under stress combinations which play a pivotal role in the stress acclimation of plants (Fig. 1).
Reactive oxygen species-abscisic acid interaction determines acclimatory stress tolerance of plants
The physiological role of ABA ranges from the maintenance of normal physiological events, like promoting dormancy in seeds, regulating stomatal conductance, and root development to stress response (Xiong et al., 2002). It is considered one of the important hormones whose biosynthesis is up-regulated under abiotic stresses (Sah et al., 2016). While ROS has a crucial role in instigating ABA-mediated signaling cascades, ABA also instigates activation of NADPH oxidase and influences the photosynthetic electron transport system (Jiang and Zhang, 2003; Zhang et al., 2006; Xia et al., 2015). There are pieces of evidence that exhibited the role of ABA in maintaining the positive feedback system for ROS production and stress tolerance by regulating the production of H2O2 (Mohanta et al., 2018). Core ABA signaling module PYR/PYL/RCAR-SnRK2-PP2C can positively regulate NADPH oxidase for ROS formation (Fig. 2). During ABA signaling, phospholipase D produces phosphatidic acid via the hydrolysis of phosphatidylcholine which binds to NADPH oxidase and activates it to produce ROS (Zhang et al., 2009) (Fig. 2). Mutation in RBOH genes causes impairment in this ABA-induced of H2O2 formation. ROS produced by NADPH oxidase generates a ROS wave. This ROS wave can induce nitrate reductase to produce NO, which in turn can increase the level of divalent calcium (Ca2+) in the cytoplasm. Ca2+ can again produce ROS by invoking RBOH with the help of calcium-dependent CPK5, CBL1-CIPK26 (Pei et al., 2000; Zhang et al., 2001; Kwak et al., 2003; Bright et al., 2006; Mittler and Blumwald, 2015; Drerup et al., 2013) (Fig. 2). There exist a positive feedback loop operating for ABA-mediated production of ROS (Raja et al., 2017). ABA signaling can transduce changes through both Ca2+-independent and Ca2+-dependent pathways. The PYR/PYL/RACR-SnRK2-PP2C module controls the Ca2+-independent pathway (Kumar et al., 2019). All PYR/PYL [pyrabactin resistance1 ((pyr1)/pyr1-like)] proteins can act as ABA receptors. These PYR/PYL/RCAR (PYR/PYL/regulatory components of aba receptors), when attached to ABA, can inhibit PP2C (type 2C protein phosphatase), which is a vital controller of calcium-independent ABA signaling (Ma et al., 2009; Park et al., 2009; Santiago et al., 2009a, 2009b; Kumar et al., 2019) (Fig. 2). Elevated level of H2O2 can inhibit these PP2C proteins (like ABI1) and thus negatively regulate ABA signaling (Fujii et al., 2009; Kumar et al., 2019) (Fig. 2). So, the ROS signaling independently influences ABA signaling through PP2C down-regulation and allowing SnRKs to down-regulate defense gene expression or inhibiting KAT1 necessary for osmolyte regulation and stomatal closure.
Figure 2: Core ABA signaling module that regulates the origin of redox cue associated with downstream signaling cascades involving NO, Ca2+, etc., in plants (detail in text).
Reactive oxygen species–C2H4 interaction determines plant performance under abiotic stress
C2H4, a gaseous phytohormone, plays a significant role in the senescence of plants, flowering, fruit ripening, nodulation, etc., and also regulates stress response under hostile environments. C2H4 invokes a prominent role in the abiotic stress tolerance of plants in particular (Reid, 1995; Lutts et al., 1996; Thompson et al., 1998; Pierik et al., 2006; Masood et al., 2012; Nazar et al., 2014; Müller and Munné-Bosch, 2015; Thao et al., 2015; Khan et al., 2017). H2O2 works downstream of C2H4 signaling pathways for exerting tolerance to salinity-stressed plants (Jiang et al., 2012, 2013). Actually, ET mediates stress response in rice through the action of the ROS signaling cascade under abiotic stresses like a flood (Steffens et al., 2013). In salinity-stressed plants, TFs like Lycopersicon esculentum ethylene responsive factor (LeERF1), LeERF2, and MsERF8 can induce the biosynthesis of proline for conferring tolerance against stress, which can also reduce oxidative membrane lipid peroxidation (Fig. 3) (Cheng et al., 2013; Hu et al., 2014). C2H4 can also reduce ROS accumulation by activating ROS detoxification or an antioxidative defense maintenance system. For example, Arabidopsis AtERF98, an ERF (Ethylene responsive factor), can give a plant tolerance against salinity stress by controlling the biosynthesis of ascorbate, which is essential for ROS detoxification via ascorbate-glutathione pathway (Zhang et al., 2012) (Fig. 3). Overexpressing mutants of CaPF1 (a TF) reduces oxidative stress by up regulating the antioxidative defense mechanism in plants in response to ROS-induced ERF activation (Youm et al., 2008) (Fig. 3). Another ERF, JERF3 regulates the transcription of many antioxidants machinery related genes like SOD, NtAPX2, APX1, glutathione peroxidase (GPx), etc. in tobacco plants in response to C2H4 signaling under salinity and drought stress, substantiating ROS-C2H4 interaction for stress tolerance (Wu et al., 2008) (Fig. 3). Under oxidative stress, the expression of ERF6 is increased and the association between ERF6 and the reduction of stress by up-regulating the antioxidant levels has been established (Fig. 3). H2O2 treatment in tobacco is associated with increased expression of TERF1, indicating the ROS-mediated expression of ERFs under different stresses. TERF1 is also associated with the up-regulation of transcription of genes like NtCAT and NtGPX to several folds, which helps to maintain the redox homeostasis needed for the alleviation of stress (Zhang et al., 2006; Sewelam et al., 2013) (Fig. 3). In a mutant of ERF6, erf6, the level of several antioxidants was reported to be low. These findings can lead to the conclusion that ERF6 is associated with the maintenance of proper antioxidant activity in stressed plants. Further, it has been seen that ERF6 is linked with the expression of MDHAR3 and CAT3 as in erf6 mutants, the level of these gene products was found to be significantly low (Fig. 3). Mutant NtCDPK2 (lacking auto-inhibitory domain) in Nicotiana bethamiana, led to generation of ROS, JA, C2H4, and 12-oxo-phytodienoic acid (Ludwig et al., 2005). However, it was noticed that elevated CDPK signaling compromised the activation of MAPK signaling through the synthesis and perception of C2H4 (Ludwig et al., 2005).
Figure 3: Role of ROS in ethylene biosynthesis and downstream signaling, showing regulation of diverse TFs associated with defense mechanism (detail in text).
Reactive oxygen species–auxin interaction determines plant development performance under abiotic stress
ROS signaling can change auxin titer in a cell through several biochemical processes, like oxidative degradation of auxin, re-localization of PIN (PIN-formed) in certain tissues, conjugation of auxins, etc. (Fig. 4) (Kawano, 2003; Raeymaekers et al., 2003; Minglin et al., 2005; Junghans et al., 2006; Park et al., 2007; Santelia et al., 2008; Tognetti et al., 2010; Grunewald and Friml, 2010; Tognetti et al., 2012). Auxin also plays an important role in responding to various abiotic stresses through interplay with ROS (Mohanta et al., 2018). It has been seen in Arabidopsis that hypocotyl cutting results in respiratory burst oxidase protein D (RBOHD) and RBOHF-dependent up-regulation of ROS production, which can increase the endogenous titer of IAA. Excised seedlings showed increased expression of YUCCA and TAA1 [(YUC) family of flavin monooxygenases/ tryptophan aminotransferase of arabidopsis 1] expression. Expression of these genes, mediated by ROS, can up-regulate the synthesis of auxin in stressed plants (von Sonntag, 1987; Armstrong, 1990; Prakash and Prathapasenan, 1990; Uchida and Kawakishi, 1993; Schoneich, 2000; Bashandy et al., 2010) (Fig. 4). In AtRbohD mutants, expression of these genes was hampered significantly. In salinity stress, tir1 (Transport inhibitor response 1) and afb2 (auxin-binding F-box protein) auxin-responsive mutants of Arabidopsis showed a significant decline in H2O2 level as compared to wild-type plants under stress. In these cases, the activities of different enzymes like ascorbate peroxidase (APX) and catalase (CAT) and transcription of glutathione S-transferase 1 (GST1) and APX1 in the mutants was enhanced, suggesting the involvement of ROS production by auxin (Iglesias et al., 2010; Mohanta et al., 2018). The level of ascorbic acid was increased significantly in mutants tir1 and afb2 (Iglesias et al., 2010). This high accumulation of ascorbic acid level may be due to the down-regulation of ascorbate oxidase (AO) activity (Bashandy et al., 2010). The lower concentration of H2O2 can be explained by the up-regulation of antioxidants. Under salinity stress, TIR1 and AFB2 activity are negatively regulated by a high level of miR393 (Fig. 4). The Arabidopsis triple mutants of ntra, ntrb, and cad2, which are involved in thioredoxin and glutaredoxin signaling-mediated redox regulation was found to affect auxin transport (Bashandy et al., 2010). Another protein of Arabidopsis, which helps in auxin-mediated stress response, is Rho-GTPase, which are products of RAC/ROP genes. ABP1, after binding with auxin, can regulate the expression of different ROP genes. These Rho-GTPase can interact with NADPH-oxidase to produce ROS in turn, which can induce several signaling cascades (Xu et al., 1994; Duan et al., 2010; Tognetti et al., 2012) (Fig. 4).
Figure 4: ROS-auxin interaction showing auxin-specific ROS signaling and subsequent modulation of endogenous titer of both the components in plants (detail in text).
Reactive oxygen species-gibberellins interaction determines germination and other developmental performances under stress
The role of GA signaling under abiotic stresses is largely regulated by DELLA proteins, which are negative regulators of GA signaling pathway. During abiotic stress, the endogenous level of GA decreases significantly, and as a result, the level of DELLA becomes high inside the cellular environment (Achard et al., 2006; Colebrook et al., 2014) (Fig. 5). GA deficit biosynthetic mutants show resistance even in severe salinity stress, whereas, plants with non-functional DELLA showed susceptibility to salt stresses. Experiments suggest that DELLA imposes tolerance to stressed plants by controlling the amount of ROS with the up-regulation of the antioxidative defense mechanism. It regulates the expression of genes expressing antioxidative enzymes and thus preventing oxidative damage in plants, thereby prolonging survival and enhancing fitness (Mohanta et al., 2018) (Fig. 5). During salt stress, DELLAs also delay ROS-induced necrosis by inhibiting their accumulation (Achard et al., 2008). In rice plants, expression of Submergence toleranace gene (Sub 1A) also restricts the ROS production through the activity of DELLA, SLR-1, and SLR-2 proteins, which are negative regulators of GA signaling (Fig. 5). This phenomenon reduces oxidative damage during the submergence of plants (Fukao et al., 2006, 2011). Superoxide dismutase (SOD) plays a pivotal role in DELLA-mediated ROS scavenging (Kliebenstein et al., 1998; Achard et al., 2008). DELLA proteins showed prominent roles in the expression of CSD1,2, which are Cu/Zn SOD encoding genes suggesting the role of GA in regulating the antioxidative defense mechanism of plants (Achard et al., 2008). Transcriptomic analysis with complete Arabidopsis microarray (CATMA) has revealed that DELLA can directly up-regulate the levels of Cu/Zn SOD, CAT, peroxidase (POD), GST1, indirectly hinting the role of GA-ROS interaction (Crowe et al., 2003; Hilson et al., 2004; Achard et al., 2008) (Fig. 5). Both exogenous GA and NaCl can significantly increase the ROS level via NADPH-oxidase activity (Apel and Hirt, 2004). In Arabidopsis, only AtrbohD was required for the production of ROS in salinity stress, and AtrbohF was not required. However, the activities of AtrbohD and AtrbohF activity are not controlled by DELLA (Achard et al., 2008). Quadruple DELLA mutants of A. thaliana also resulted in reduced ROS accumulation and enhanced expression of antioxidant enzymes-producing genes during salinity stress (Achard et al., 2009). In maize also, reduced GA levels and enhanced DELLA activity were observed during dehydration stress, which facilitated ROS scavenging (Wang et al., 2008). It is also noticed that GA2ox7 (GA 2-oxidase) can reduce the level of bioactive GA under salinity stress by upregulating the synthesis of the C20-GA deactivating enzyme (Magome et al., 2008). Another experiment by Shan et al. (2014) revealed that overexpressed GA2ox5 conferred high salt tolerance to plants but reduced GA levels (Shan et al., 2014). GA20ox3 is an important enzyme in GA biosynthetic pathway that helps in bioactive GA4 synthesis. Tudor-SN (TSN), a very common protein of Arabidopsis, also plays important role in the maintenance of stress by controlling the level of GA20ox3 mRNA. So, by modulating GA20ox3 and TSN, one can reduce the concentration of bioactive GA, which in turn can confer greater stress tolerance (Yan et al., 2014) (Fig. 5). On the contrary, ROS produced during different abiotic stresses can induce GA synthesis with the help of enzymes like GA3ox (Kai et al., 2016) (Fig. 5). Therefore, the impact of ROS-GA interaction seems to be flexible depending on the signaling module and environmental stress, which ultimately regulate plant performance under stress.
Figure 5: ROS-GA interaction showing the involvement of DELLA, NADPH-oxidase and other TFs in regulating physiology of plants under stress (detail in text).
Reactive oxygen species-salicylic acid interaction determines stress tolerance and the developmental process of plants
One of the key phytohormones, SA, confers tolerance to both abiotic and biotic stresses to plants (Drzewiecka et al., 2012; Li et al., 2017; Thevenet et al., 2017). Exogenous application or endogenous biosynthesis of SA and accumulation both help in tolerance to abiotic stress like metal, salinity, drought, high temperature, ozone, etc. (Khan et al., 2015). Plants under salinity stress can show better tolerance to stress with the help of SA-mediated up-regulation of different stress-tolerant mechanisms (Azooz, 2009; Nazar et al., 2011; Palma et al., 2013; Nazar et al., 2014, 2015). ROS plays an important role in SA-mediated responses during abiotic stress and acts directly or indirectly on different stress tolerance mechanisms (Lee and Park, 2010; Herrera-Vásquez et al., 2015). During the early event of stress signaling, SA promotes ROS signaling using peroxidase (PRX) and acts as a key player in the plant defense system (Lee and Park, 2010; Garretón et al., 2002; Khokon et al., 2011; Miura et al., 2013). It has been found that ROS signaling, initiates primarily at the chloroplast of the guard cell under the influence of SA. SA is suggested to be associated with ROS generation in chloroplasts and peroxisomes (Apel and Hirt, 2004; Joo et al., 2005; Herrera-Vásquez et al., 2015). However, the exact mechanism of H2O2 production via SA signaling in the apoplast, peroxisome, and chloroplast remains unknown. Also, ICS1 (Isochorismate synthase 1) regulating transcription factors like SARD1,CBP60,WRKY8/28/48, CAMTA 3/SR1, and ZAT6 is associated with ROS-mediated signaling and biosynthesis of SA (Du et al., 2009; Zhang et al., 2010a; van Verk et al., 2011; Shi et al., 2014) (Fig. 6). During different stresses, the redox homeostasis of plant cell is disrupted that causes overaccumulation of ROS, which in turn instigates oxidative damage of different cellular components (Mittler, 2002; Torres et al., 2002; Miller et al., 2008). SA helps to decrease the electron leakage from the photosynthetic electron transport chain and inhibits ROS generation (Khan et al., 2014). Again, SA can protect cells from oxidative damage caused by ROS by up-regulating different antioxidative defense mechanisms like APX, SOD, Glutathione peroxidase (GPO), glutathione peroxidase (GR), etc. (Janda et al., 1999; Kang et al., 2013; Li et al., 2013) (Fig. 6). Different metal tolerant plants like Linum usitatissimum are tolerant to metal stress-induced oxidative stress due to SA-mediated detoxification of ROS like H2O2 (Belkadhi et al., 2015) (Fig. 6). Application of exogenous SA in metal stressed plants have shown up-regulation of different enzymatic and non-enzymatic antioxidants, which helps to reduce lipid peroxidation due to ROS (Jing et al., 2007; Zengin, 2014). Plants with heavy metal accumulation ability are associated with a high concentration of SA biosynthesis components and derivatives of those components, indicating that the biosynthesis of SA is up-regulated in those heavy metal hyperaccumulators (Freeman et al., 2005). A proteomics study has revealed that SA treatment can enhance the expression of at least 37 proteins under drought stress. These proteins include antioxidants like GST, APX, etc., which can work for maintaining redox homeostasis. SA increases transcription of different antioxidant genes like GPX1/2, dehydroascorbate reductase (DHAR), mono-dehydroascorbate reductase (MDHAR), GST1/2, etc., under different abiotic stresses that strengthen the antioxidative mechanism and help to protect plants from environmental odds. Application of SA on plants under drought stress has also shown increased transcription of chaperones and heat shock proteins (HSPs) (Fig. 6). This indicates that SA can help in proper protein folding under stress. Plants under drought stress even can increase the biosynthesis of different secondary metabolites like lignin-related cinnamyl alcohol dehydrogenase (SAD, CAD) and cryptochrome P450 for fighting against stress (Jumali et al., 2011) (Fig. 6). It is assumed that MAPK cascades play an important role in SA-mediated abiotic stress tolerance by up-regulating transcription of different genes. MAP (Mitogen-activated protein) kinases like MPK3/4/6 play a crucial role in abiotic stress tolerance and are important components for SA-ROS signaling (Baier et al., 2005; Kangasjärvi et al., 2005; Fujita et al., 2006). MEKK1, which is a kind of MAP kinase kinase, can be directly controlled by SA and ROS (Fujita et al., 2006; Suarez-Rodriguez et al., 2007). Two MAP kinases, MPK6 and MPK3 in Arabidopsis, can be controlled under the influence of MAP kinase kinase proteins like MKK4 and MKK5 (Baier et al., 2005). MPK4 is seen to be activated in those plants, where the MPK6 is mutated; but under normal conditions, MPK6 is not associated with active MPK4. This indicates that MPK6 inhibits MPK4 activity in Arabidopsis in SA-mediated stress signaling, and there must be two different MAPK signaling cascades working together under the influence of MEKK1 (Menke et al., 2004). Under the influence of these MAPK cascades, different antioxidant genes (SOD, HSP, APX, POD) are expressed, substantiating ROS-SA interaction, which helps to tolerate abiotic stress (Chini et al., 2004; Gruhler et al., 2005; Salzman et al., 2005; Rajjou et al., 2006) (Fig. 6). However, it has been reported that treatments with high concentrations (>100 μM) of SA cause higher SA production in plants leading to an oxidative burst and reduced tolerance to drought and salinity-stresses (Lee et al., 2010; Miura and Tada, 2014).
Figure 6: ROS-SA interaction in plant cell showing SA-induced changes in redox homeostasis, antioxidative defense and morphogenesis (detail in text).
SA has also been found to enhance adventitious root formation (ARF) via increasing endogenous titer of ROS (H2O2) (Babar Ali et al., 2007; Yang et al., 2013; Kora and Bhattacharjee, 2020). Explants incubated in SA after primary root removal showed the maximum concentration of H2O2 at 12 h. Combined treatment of SA and H2O2 resulted in higher adventitious roots than their individual treatment in mung bean explants (Yang et al., 2013; Kora and Bhattacharjee, 2020). SA treatment down-regulates CAT activity and activities of enzymes of the central redox hub, the Halliwell Asada pathway (APX, DHAR GR), and simultaneously increases the activity of SOD and RBOH (NADPH-oxidase), suggesting the important role of ROS as a second messenger in SA-mediated ARF (Yang et al., 2013; Kora and Bhattacharjee, 2020) (Fig. 6).
Reactive oxygen species-Brassinosteroid (BR) interaction in plant cell under stress
BR triggers many stress signaling pathways with the help of Ca2+ and ROS as a second messenger (Allen et al., 2000). In response to stress, BR can regulate the ROS titer by modulating ROS generation as well as the ROS scavenging mechanism, thus changing the redox status of the cell for precise regulation of redox signaling for stress adaptations (Jakubowska and Janicka, 2017; Ahmad et al., 2018). BR induces NADPH-oxidase-mediated ROS generation in the apoplast, and silencing of RBOH1 reduces BR-mediated ROS production (Nie et al., 2013). MAPK cascades play an important role in BR-dependent ROS production (Zhang et al., 2010b) (Fig. 7). A microtubule-associate protein MAP65-1a, whose transcription is dependent on BR, turns MAPK cascades on, which ultimately regulates the expression of NADPH-oxidase genes (Zhu et al., 2013) (Fig. 7). Experiments have proven that NADPH-oxidase activity is confluent with the activity of endogenous BR level (Xia et al., 2009). Overly expressed BR biosynthesis gene CYP1 leads to ROS production and change in the redox status, which in turn produces ABA and evokes stress response. Biosynthesis modulation of BR is also observed by feedback repression of BR biosynthesis genes (Bancosİ et al., 2002). BR, along with ABA, plays an important role in stomatal regulation that includes ROS (Fig. 7). Low BR level is associated with ephemeral ROS production, which leads to stomatal opening, whereas a high BR level induces prolonged ROS generation through NADPH-oxidase activity which is subsequently associated with high ABA level and leads to stomatal closure (Xia et al., 2014; Mohanta et al., 2018) (Fig. 7). ABA might also induce NO synthesis along with ROS. Both of these can induce 8-nitro-cGMP, which promotes stomatal closing during abiotic stress conditions (Joudoi et al., 2013). JA produced during stressed conditions interacts with ABA-mediated stomatal closure by stimulating the extracellular Ca2+influx and/or by activating H2O2/NO signaling pathways (Fig. 8). On the contrary, another finding by Desikan et al. (2006) provided much evidence that stomatal closure by ethylene is regulated via its signal transduction pathway, which stimulates the production of H2O2 and requires H2O2 synthesis (Fig. 8). Treatment with either ABA or methyl jasmonate (MeJA) for 10 min resulted in a reduction of stomatal aperture in turgid and excised leaves of Arabidopsis and suggested both ABA and MeJA interact in guard cells and induce the formation of ROS and NO (Wani et al., 2016).
Figure 7: ROS-BR synergistic action in plant cell showing BR-induced induction of redox, NO and ABA signaling regulating stomatal conductance and plant defense processes (detail in text).
Figure 8: ROS-JA interaction under stress in plant cell showing both feed-forward and feed-backward and interactions for influencing their endogenous titres and regulation of stress tolerance mechanism (detail in text).
ABA levels in the BR biosynthetic mutants are found to be low, but the exogenous application of BR on the mutant might raise ABA levels (Zhou et al., 2014). These findings suggest a significant link between ABA, GA, BRs, and ROS during abiotic stress in plants. The application of BR biosynthesis inhibitor brassinazole (BRZ) decreases the endogenous level of BR, which leads to decreased tolerance to different abiotic stresses (Xia et al., 2009). Constitutive BR signaling mutant, bes1-D, resulted in constitutive activation of BR signaling that leads to stress tolerance in plants (Sun et al., 2010). Kim et al. (2012) showed that BR could induce MAPK cascades by inhibiting BIN2 (BR-INSENSITIVE 2) and inhibition (Fig. 7). Silencing genes like RBOH1, MPK2 or MPK1/2 caused inhibition of BR-mediated stress signaling to some extent (Nie et al., 2013). Silencing of MPK2 can give greater silencing of BR-mediated signaling than MPK1. This suggests that MPK2 plays a more important role as compared to MPK1 during stress tolerance. Also, MPK1/2 is seen to play an important role in ROS generation, and MPK2, ROS, and RBOH1 are assumed to work together in a positive feedback loop for facilitating BR-mediated signaling for environmental stress tolerance (Zhou et al., 2014). MAPK cascades in plants can positively up-regulate many defenses related genes like Cu-Zn SOD, GR1, APX1, CAT1, NPR1, PR1, WRKY1 even HSPs like HSP90, in which transcription factors like WRKY plays an important role in conferring tolerance to plants under stress (Zhou et al., 2014) (Fig. 7). Transcription factors like WRKY46, 54, and 70 assist in BR-mediated stress signaling with the help of Brassinosteroid signaling positive regulator (BZR1) family protein (BES1) (Banerjee and Roychoudhury, 2015; Chen and Yin, 2017) (Fig. 7).
Reactive oxygen species-jasmonate interaction in plant cells under stress
JA synthesis happens to be involved in wound and stress response. During mechanical injury, Ca2+ channels sense mechanical shear, and along with other signaling components, it induces several MAPK cascades that involve NADPH-oxidase for JA biosynthesis (Wolf et al., 2012) (Fig. 8). Oligogalacturonides, which are produced from wound stress, can prompt the biosynthesis of JA (Doares et al., 1995) (Fig. 8). Abiotic stresses like cold stress induces up-regulation of transcription of different JA biosynthetic genes like AOC, AOS1, and LOX2, leading to high accumulation of JA in the cold stressed plants (Hu et al., 2017) (Fig. 8). The level of JA is increased initially during drought stress but in prolonged drought stress the level drops to a normal level as seen in unstressed plants (Wang et al., 2020). Wounding or other abiotic stress can alter the redox status of cells, leading to the generation of different ROS in plants which is assumed to be associated with JA biosynthesis in plants (Parra-Lobato et al., 2009) (Fig. 8). In return, JA helps in maintaining physiological responses under abiotic stress by activation of antioxidant machinery, accumulation of different amino acids like proline, accumulation of sugars, and regulation of stomatal conductance as a general response (Acharya and Assmann, 2009; Karpets et al., 2014; Wasternack, 2014) (Fig. 8). On the other hand, Endogenous JA modulates the titer of ROS associated with salinity stress tolerance. It also increases the salinity stress tolerance by increasing photosynthetic rate, ABA concentration, proline content, the activity of enzymatic antioxidants, etc. (Bandurska et al., 2003; Walia et al., 2007; Khan et al., 2012; Abouelsaad and Renault, 2018) (Fig. 8). Qiu et al. (2014) showed JA-indued significant oxidative damage in salt-stressed wheat seedlings via up-regulation of gene expression of SOD, POD, CAT, and APX genes, indicating the role of JA in redox regulation (Qiu et al., 2014) (Fig. 8). Exogenous application of MeJA could alleviate the adverse consequences of drought stress by up-regulating different enzymatic antioxidants like POD, SOD, CAT, APX, GR, and non-enzymatic antioxidants like proline, soluble sugar, etc. (Wu et al., 2012). Several other studies done on different stressed plants have shown that JA and MeJA help to withstand by up-regulating the antioxidative defense mechanism and maintaining the redox status of the cell (Soares et al., 2010; Manar et al., 2013; Faghih et al., 2017). Also, a very high level of JA seems to be associated with ROS accumulation in cells and programmed cell death under stress (Orozco-Cárdenas and Ryan, 2002; Xia et al., 2015) (Fig. 8). Takahashi et al. (2007) have shown that in JA mediated stress signaling, MAPK cascade plays a significant role and MKK3 and MPK6 are the most important components in that signaling cascade.
ROS is an intrinsic part of the signaling network that plays a major role in the responses against abiotic stress in plants. Evidences are accumulating that suggests that ROS-hormone interplay forms an integrated signaling web that can act to attenuate environmental stresses. In those pathways, ROS itself can act as a signaling molecule or influence other bona fide hormonal signaling modules by changing the redox status of cells. ROS can modulate hormone biosynthesis and or influence hormonal signaling circuits. ROS can directly up-regulate several enzymes for the biosynthesis of phytohormones, and can also regulate the endogenous titer of several hormones by influencing their degradation or chelation for proper response under stress. These chemically reactive molecules are also involved in oxidative degradation and localization of various hormones for proper stress response of plants. ROS can also mediate hormone-hormone interaction under several situations. The reverse events are also noticed, wherein hormones can up-regulate the synthesis of ROS and subsequently recruit them as ‘second messenger’ in several stress acclamatory signaling events. ROS can operate several common MAPK cascades for different hormones; also, it can turn on hormone-specific MAPK cascades depending on the environmental stimulus. Gene expression in response to a hormone signaling up-regulates the expression of several stress-specific genes. Many important TFs like WRKY (WRKY 1, 46, 54, 70), ZAT, NAC, etc., are expressed as a result of redox-hormone interaction. Also, the transcription of many chaperones and HSPs are positively regulated by redox-hormone signaling.
As previously discussed, one of the cornerstones of starting these signaling cascades by various phytohormones and ROS itself involves a change in the redox status of cells under several environmental odds. These signaling cascades shoots up, and the ultimate motto of the signaling web is to bring the cell back to its original state where it can function properly, i.e., perform the normal physiological activity. Different ROS scavenging machinery, along with other responsive factors, gets turned on to alleviate stress and help to perform physiological functions. Though the interaction between ROS-hormones is elucidated to some extent, detailed ROS-dependent hormone signaling pathways need to be deciphered for greater understanding to give us a clear picture of ROS-mediated converging pathways of hormone signaling under abiotic stress.
The present review tried to explore the central role of ROS–hormone interaction in defense signaling under environmental threats. The well-characterized role of redox signaling coupled with Ca++ signaling with downstream kinase and phosphatase activities that form flexible, interactive roles were found to be extremely important and necessary for the amplification of signaling response and survival of plants under stress. The redox regulation of several molecular events, like activation of transcription factors, transporter proteins, enzymes activation associated with hormonal metabolism and signaling, etc., have been found to perform a key role in stress defense and developmental processes. In spite of all these evidences, the most challenging aspect of the ROS-hormone integrative defense signaling pathway is understanding this aspect from the point of view of stress combinations, where different individual specific signaling molecules interact and collide. Further, it will be more challenging to explore ROS–hormone interaction in view of the conflict of interest that arise under different stress combinations. Moreover, the mechanistic aspect of the spatio-temporal regulation of ROS and their relative subcellular activities to control and regulate hormonal signaling is yet to be understood. The technical backup needed to prove the detail of molecular redox regulatory events (redox impacted modification of target molecules and their functional changes) needs to be explored further.
In spite of unprecedented progress in plant redox biology and hormonal cell signaling process, the precise position of these two highly dynamic signaling components in the integrative signaling web largely remains obscured and hence necessitates further molecular genetic investigation along with physiological study for ascertaining their exact status, position, and the function in the plant cell. Taken as a whole, the process of redox regulation through ROS production and processing is found to be an integral part of hormonal physiology and the functioning of plant cells, and there exists a very complicated interactive signaling mesh to accomplish this function. Our understanding regarding this complex signaling network between ROS–hormone signaling is thus far from complete.
Acknowledgement: We apologize sincerely if we inadvertently omitted citations of contributors to the area of plant redox biology. We also thank the reviewers for their comments.
Author Contribution: Conceptualization S.B., Original draft preparation S.B., D.K., Manuscript and figures: D.K., N.D., U.K.R., D.S., M.A., A.D., B.P., A.K. and T.B. All authors read and approved the final manuscript.
Ethics Approval: Not applicable.
Funding Statement: S.B. acknowledges DST-SERB (Government of India) for research funding (No. CRG/2021/000513, dated 15/12/2021). All authors also acknowledge UGC-CAS and DST-FIST (Government of India) for infrastructural support for research to the Department of Botany, University of Burdwan, India [No. F.5-13/012 (SAP-II), and No.SRFST/LS-I/2018/188 (C)]. D.K., A.D., B.P. and A.K. acknowledge the University Grants Commission (UGC), New Delhi, for Junior Research Fellowship (Joint CSIR-UGC). N.D. acknowledges the State Funded Research Grant, Government of West Bengal. India [No.FC(Sc.)/RS/SF/BOT/2016-17/210/1(4)]. D.S. acknowledges Department of Science Technology and Biotechnology (DSTBT), Government of West Bengal. India. U.K.R. acknowledges Indian Council for Cultural Relations (ICCR) for India Scholarships (Bangladesh) Scheme, 2016-2017 (No. DAC/EDU/17/1/2016, dated 10.07.2016). T.B. acknowledges DST-SERB, Government of India.
Conflicts of Interest: The authors declare that they have no conflicts of interest to report regarding the present study.
References
Abouelsaad I, Renault S (2018). Enhanced oxidative stress in the jasmonic acid-deficient tomato mutant def-1 exposed to NaCl stress. Journal of Plant Physiology 226: 136–144. DOI 10.1016/j.jplph.2018.04.009. [Google Scholar] [CrossRef]
Achard P, Cheng H, de Grauwe L, Decat J, Schoutteten H, et al. (2006). Integration of plant responses to environmentally activated phytohormonal signals. Science 311: 91–94. DOI 10.1126/science.1118642. [Google Scholar] [CrossRef]
Achard P, Gusti A, Cheminant S, Alioua M, Dhondt S, Coppens F, Beemster GTS, Genschik P (2009). Gibberellin signaling controls cell proliferation rate in Arabidopsis. Current Biology 19: 1188–1193. DOI 10.1016/j.cub.2009.05.059. [Google Scholar] [CrossRef]
Achard P, Renou JP, Berthomé R, Harberd NP, Genschik P (2008). Plant DELLAs restrain growth and promote survival of adversity by reducing the levels of reactive oxygen species. Current Biology 18: 656–660. DOI 10.1016/j.cub.2008.04.034. [Google Scholar] [CrossRef]
Acharya BR, Assmann SM (2009). Hormone interactions in stomatal function. Plant Molecular Biology 69: 451–462. DOI 10.1007/s11103-008-9427-0. [Google Scholar] [CrossRef]
Ahmad F, Singh A, Kamal A (2018). Crosstalk of brassinosteroids with other phytohormones under various abiotic stresses. Journal of Applied Biology and Biotechnology 6: 56–62. DOI 10.7324/JABB.2018.60110. [Google Scholar] [CrossRef]
Allen GJ, Chu SP, Schumacher K, Shimazaki CT, Vafeados D et al. (2000). Alteration of stimulus-specific guard cell calcium oscillations and stomatal closing in Arabidopsis det3 mutant. Science 289: 2338–2342. DOI 10.1126/science.289.5488.2338. [Google Scholar] [CrossRef]
Anjum NA, Khan NA, Sofo A, Baier M, Kizek R (2016). Redox homeostasis managers in plants under environmental stresses. Frontiers in Environmental Science 35: 113. DOI 10.3389/fenvs.2016.00035. [Google Scholar] [CrossRef]
Apel K, Hirt H (2004). Reactive oxygen species: Metabolism, oxidative stress, and signal transduction. Annual Review of Plant Biology 55: 373–399. DOI 10.1146/annurev.arplant.55.031903.141701. [Google Scholar] [CrossRef]
Armstrong DA (1990). Applications of pulse radiolysis for the study of short-lived sulphur species. In: Sulfur-Centered Reactive Intermediates in Chemistry and Biology, pp. 121-134. Boston: Springer. [Google Scholar]
Azooz MM (2009). Salt stress mitigation by seed priming with salicylic acid in two faba bean genotypes differing in salt tolerance. International Journal of Agriculture and Biology 11: 343–350. [Google Scholar]
Babar Ali M, Hahn EJ, Paek KY (2007). Methyl jasmonate and salicylic acid induced oxidative stress and accumulation of phenolics in Panax ginseng bioreactor root suspension cultures. Molecules 12: 607–621. DOI 10.3390/12030607. [Google Scholar] [CrossRef]
Baier M, Kandlbinder A, Golldack D, Dietz KJ (2005). Oxidative stress and ozone: Perception, signalling and response. Plant, Cell & Environment 28: 1012–1020. DOI 10.1111/pce.2005.28.issue-8. [Google Scholar] [CrossRef]
Balfagόn D, Sengupta S, Gomez-Cadenas A, Fritschi FB, Azad R, Mittler R, Zandalinas SI (2019). Jasmonic acid is required for plant acclimation to a combination of high light and heat stress. Plant Physiology 181: 1668–1682. DOI 10.1104/pp.19.00956. [Google Scholar] [CrossRef]
Bancosİ S, Nomura T, Sato T, Molnár G, Bishop GJ, Koncz C, Yokota T, Nagy F, Szekeres M (2002). Regulation of transcript levels of the Arabidopsis cytochrome P450 genes involved in brassinosteroid biosynthesis. Plant Physiology 130: 504–513. DOI 10.1104/pp.005439. [Google Scholar] [CrossRef]
Bandurska H, Stroiński A, Kubiś J (2003). The effect of jasmonic acid on the accumulation of ABA, proline and spermidine and its influence on membrane injury under water deficit in two barley genotypes. Acta Physiologiae Plantarum 25: 279–285. DOI 10.1007/s11738-003-0009-0. [Google Scholar] [CrossRef]
Banerjee A, Roychoudhury A (2015). WRKY proteins: Signaling and regulation of expression during abiotic stress responses. The Scientific World Journal 2015: 1–17. DOI 10.1155/2015/807560. [Google Scholar] [CrossRef]
Bartoli CG, Casalongué CA, Simontacchi M, Marquez-Garcia B, Foyer CH (2013). Interactions between hormone and redox signalling pathways in the control of growth and cross tolerance to stress. Environmental and Experimental Botany 94: 73–88. DOI 10.1016/j.envexpbot.2012.05.003. [Google Scholar] [CrossRef]
Bashandy T, Guilleminot J, Vernoux T, Caparros-Ruiz D, Ljung K, Meyer Y, Reichheld J-P (2010). Interplay between the NADP-linked thioredoxin and glutathione systems in Arabidopsis auxin signaling. The Plant Cell 22: 376–391. DOI 10.1105/tpc.109.071225. [Google Scholar] [CrossRef]
Belkadhi A, De Haro A, Obregon S, Chaïbi W, Djebali W (2015). Positive effects of salicylic acid pretreatment on the composition of flax plastidial membrane lipids under cadmium stress. Environmental Science and Pollution Research 22: 1457–1467. DOI 10.1007/s11356-014-3475-6. [Google Scholar] [CrossRef]
Bhattacharjee S (2012). The language of reactive oxygen species signaling in plants. Journal of Botany 2012. DOI 10.1155/2012/985298. [Google Scholar] [CrossRef]
Bhattacharjee S (2019). ROS and oxidative stress: Origin and implication. In: Reactive Oxygen Species in Plant Biology, pp. 1–31. New Delhi: Springer. [Google Scholar]
Bright J, Desikan R, Hancock JT, Weir IS, Neill SJ (2006). ABA-induced NO generation and stomatal closure in Arabidopsis are dependent on H2O2 synthesis. The Plant Journal 45: 113–122. DOI 10.1111/j.1365-313X.2005.02615.x. [Google Scholar] [CrossRef]
Chen Z, Silva H, Klessig DF (1993). Active oxygen species in the induction of plant systemic acquired resistance by salicylic acid. Science 262: 1883–1886. DOI 10.1126/science.8266079. [Google Scholar] [CrossRef]
Chen J, Yin Y (2017). WRKY transcription factors are involved in brassinosteroid signaling and mediate the crosstalk between plant growth and drought tolerance. Plant Signaling & Behavior 12: e1365212. DOI 10.1080/15592324.2017.1365212. [Google Scholar] [CrossRef]
Cheng MC, Liao PM, Kuo WW, Lin TP (2013). The Arabidopsis ETHYLENE RESPONSE FACTOR1 regulates abiotic stress-responsive gene expression by binding to different cis-acting elements in response to different stress signals. Plant Physiology 162: 1566–1582. DOI 10.1104/pp.113.221911. [Google Scholar] [CrossRef]
Chini A, Grant JJ, Seki M, Shinozaki K, Loake GJ (2004). Drought tolerance established by enhanced expression of the CC-NBS–LRR gene, ADR1, requires salicylic acid, EDS1 and ABI1. The Plant Journal 38: 810–822. DOI 10.1111/j.1365-313X.2004.02086.x. [Google Scholar] [CrossRef]
Choudhury FK, Rivero RM, Blumwald E, Mittler R (2017). Reactive oxygen species, abiotic stress and stress combination. Plant Journal 90: 856–867. DOI 10.1111/tpj.13299. [Google Scholar] [CrossRef]
Colebrook EH, Thomas SG, Phillips AL, Hedden P (2014). The role of gibberellin signalling in plant responses to abiotic stress. Journal of Experimental Biology 217: 67–75. DOI 10.1242/jeb.089938. [Google Scholar] [CrossRef]
Crowe ML, Serizet C, Thareau V, Aubourg S, Rouze P et al. (2003). CATMA: A complete Arabidopsis GST database. Nucleic Acids Research 31: 156–158. DOI 10.1093/nar/gkg071. [Google Scholar] [CrossRef]
Desikan R, Last K, Harrett-Williams R, Tagliavia C, Harter K, Hooley R, Hancock JT, Neill SJ (2006). Ethylene-induced stomatal closure in Arabidopsis occurs via AtrbohF-mediated hydrogen peroxide synthesis. Plant Journal 47: 907–916. DOI 10.1111/j.1365-313X.2006.02842.x. [Google Scholar] [CrossRef]
Devireddy RA, Zandalinas SI, Fichman Y, Mittler R (2021). Integration of reactive oxygen species and hormone signaling during abiotic stress. The Plant Journal 105: 459–476. DOI 10.1111/tpj.15010. [Google Scholar] [CrossRef]
Doares SH, Syrovets T, Weiler EW, Ryan CA (1995). Oligogalacturonides and chitosan activate plant defensive genes through the octadecanoid pathway. PNAS 92: 4095–4098. DOI 10.1073/pnas.92.10.4095. [Google Scholar] [CrossRef]
Drerup MM, Schlücking K, Hashimoto K, Manishankar P, Steinhorst L et al. (2013). The calcineurin B-like calcium sensors CBL1 and CBL9 together with their interacting protein kinase CIPK26 regulate the Arabidopsis NADPH oxidase RBOHF. Molecular Plant 6: 559–569. DOI 10.1093/mp/sst009. [Google Scholar] [CrossRef]
Drzewiecka K, Borowiak K, Bandurska H, Golinski P (2012). Salicylic acid—A potential biomarker of tobacco Bel-W3 cell death developed as a response to ground level ozone under ambient conditions. Acta Biologica Hungarica 63: 231–249. DOI 10.1556/ABiol.63.2012.2.6. [Google Scholar] [CrossRef]
Du L, Ali GS, Simons KA, Hou J, Yang T, Reddy AS, Poovaiah BW (2009). Ca 2+/calmodulin regulates salicylic-acid-mediated plant immunity. Nature 457: 1154–1158. DOI 10.1038/nature07612. [Google Scholar] [CrossRef]
Duan Q, Kita D, Li C, Cheung AY, Wu HM (2010). FERONIA receptor-like kinase regulates RHO GTPase signaling of root hair development. PNAS 107: 17821–17826. DOI 10.1038/nature07612. [Google Scholar] [CrossRef]
Faghih S, Ghobadi C, Zarei A (2017). Response of strawberry plant cv. ‘Camarosa’ to salicylic acid and methyl jasmonate application under salt stress condition. Journal of Plant Growth Regulation 36: 651–659. DOI 10.1007/s00344-017-9666-x. [Google Scholar] [CrossRef]
Fang P, Yan M, Chi C, Wang M, Zhou Y, Zhou J, Shi K, Xia X, Foyer CH, Yu J (2019). Brassinosteroids act as a positive regulator of photoprotection in response to chilling stress. Plant Physiology 180: 2061–2076. DOI 10.1104/pp.19.00088. [Google Scholar] [CrossRef]
Fichman Y, Mittler R (2020). Rapid systemic signaling during abiotic and biotic stresses: Is the ROS wave master of all trades? The Plant Journal 102: 887–896. DOI 10.1111/tpj.14685. [Google Scholar] [CrossRef]
Foyer CH, Noctor G (2013). Redox signaling in plants. Antioxidants & Redox Signaling 18: 2087–2090. DOI 10.1089/ars.2013.5278. [Google Scholar] [CrossRef]
Freeman JL, Garcia D, Kim D, Hopf A, Salt DE (2005). Constitutively elevated salicylic acid signals glutathione-mediated nickel tolerance in Thlaspi nickel hyperaccumulators. Plant Physiology 137: 1082–1091. DOI 10.1104/pp.104.055293. [Google Scholar] [CrossRef]
Fujii H, Chinnusamy V, Rodrigues A, Rubio S, Antoni R, Park SY, Cutler SR, Sheen J, Rodriguez PL, Zhu JK (2009). In vitro reconstitution of an abscisic acid signalling pathway. Nature 462: 660–664. DOI 10.1038/nature08599. [Google Scholar] [CrossRef]
Fujita M, Fujita Y, Noutoshi Y, Takahashi F, Narusaka Y, Yamaguchi-Shinozaki K, Shinozaki K (2006). Crosstalk between abiotic and biotic stress responses: A current view from the points of convergence in the stress signaling networks. Current Opinion in Plant Biology 9: 436–442. DOI 10.1016/j.pbi.2006.05.014. [Google Scholar] [CrossRef]
Fukao T, Xu K, Ronald PC, Bailey-Serres J (2006). A variable cluster of ethylene response factor-like genes regulates metabolic and developmental acclimation responses to submergence in rice. The Plant Cell 18: 2021–2034. DOI 10.1105/tpc.106.043000. [Google Scholar] [CrossRef]
Fukao T, Yeung E, Bailey-Serres J (2011). The submergence tolerance regulator SUB1A mediates crosstalk between submergence and drought tolerance in rice. The Plant Cell 23: 412–427. DOI 10.1105/tpc.110.080325. [Google Scholar] [CrossRef]
Garretón V, Carpinelli J, Jordana X, Holuigue L (2002). The as-1 promoter element is an oxidative stress-responsive element and salicylic acid activates it via oxidative species. Plant Physiology 130: 1516–1526. DOI 10.1104/pp.009886. [Google Scholar] [CrossRef]
Gruhler A, Schulze WX, Matthiesen R, Mann M, Jensen ON (2005). Stable isotope labeling of Arabidopsis thaliana cells and quantitative proteomics by mass spectrometry. Molecular & Cellular Proteomics 4: 1697–1709. DOI 10.1074/mcp.M500190-MCP200. [Google Scholar] [CrossRef]
Grunewald W, Friml J (2010). The march of the PINs: Developmental plasticity by dynamic polar targeting in plant cells. The EMBO Journal 29: 2700–2714. DOI 10.1038/emboj.2010.181. [Google Scholar] [CrossRef]
Herrera-Vásquez A, Salinas P, Holuigue L (2015). Salicylic acid and reactive oxygen species interplay in the transcriptional control of defense genes expression. Frontiers in Plant Science 6: 171. DOI 10.3389/fpls.2015.00171. [Google Scholar] [CrossRef]
Hilson P, Allemeersch J, Altmann T, Aubourg S, Avon A et al. (2004). Versatile gene-specific sequence tags for Arabidopsis functional genomics: Transcript profiling and reverse genetics applications. Genome Research 14: 2176–2189. DOI 10.1101/gr.2544504. [Google Scholar] [CrossRef]
Hu Y, Jiang Y, Han X, Wang H, Pan J, Yu D (2017). Jasmonate regulates leaf senescence and tolerance to cold stress: Crosstalk with other phytohormones. Journal of Experimental Botany 68: 1361–1369. DOI 10.1093/jxb/erx004. [Google Scholar] [CrossRef]
Hu N, Tang N, Yan F, Bouzayen M, Li Z (2014). Effect of LeERF1 and LeERF2 overexpression in the response to salinity of young tomato (Solanumlycopersicum cv. Micro-Tom) seedlings. Acta Physiologiae Plantarum 36: 1703–1712. DOI 10.1007/s11738-014-1545-5. [Google Scholar] [CrossRef]
Iglesias MJ, Terrile MC, Bartoli CG, D’Ippólito S, Casalongué CA (2010). Auxin signaling participates in the adaptative response against oxidative stress and salinity by interacting with redox metabolism in Arabidopsis. Plant Molecular Biology 74: 215–222. DOI 10.1007/s11103-010-9667-7. [Google Scholar] [CrossRef]
Jakubowska D, Janicka M (2017). The role of brassinosteroids in the regulation of the plasma membrane H+-ATPase and NADPH oxidase under cadmium stress. Plant Science 264: 37–47. DOI 10.1016/j.plantsci.2017.08.007. [Google Scholar] [CrossRef]
Janda T, Szalai G, Tari I, Paldi E (1999). Hydroponic treatment with salicylic acid decreases the effects of chilling injury in maize (Zea mays L.) plants. Planta 208: 175–180. DOI 10.1007/s004250050547. [Google Scholar] [CrossRef]
Jiang C, Belfield EJ, Cao Y, Smith JAC, Harberd NP (2013). An Arabidopsis soil-salinity-tolerance mutation confers ethylene-mediated enhancement of sodium/potassium homeostasis. Plant Cell 25: 3535–3552. DOI 10.1105/tpc.113.115659. [Google Scholar] [CrossRef]
Jiang C, Belfield EJ, Mithani A, Visscher A, Ragoussis J, Mott R, Smith JA, Harberd NP (2012). ROS-mediated vascular homeostatic control of root-to-shoot soil Na delivery in Arabidopsis. The EMBO Journal 31: 4359–4370. DOI 10.1038/emboj.2012.273. [Google Scholar] [CrossRef]
Jiang M, Zhang J (2003). Cross-talk between calcium and reactive oxygen species originated from NADPH oxidase in abscisic acid-induced antioxidant defence in leaves of maize seedlings. Plant, Cell & Environment 26: 929–939. DOI 10.1046/j.1365-3040.2003.01025.x. [Google Scholar] [CrossRef]
Jing CH, Cheng ZH, Li LP, Sun ZY, Pan XB (2007). Effects of exogenous salicylic acid on growth and H2O2-metabolizing enzymes in rice seedlings under lead stress. Journal of Environmental Sciences 19: 44–49. DOI 10.1016/S1001-0742(07)60007-2. [Google Scholar] [CrossRef]
Joo JH, Wang S, Chen JG, Jones AM, Fedoroff NV (2005). Different signaling and cell death roles of heterotrimeric G protein α and β subunits in the Arabidopsis oxidative stress response to ozone. Plant Cell 17: 957–970. DOI 10.1105/tpc.104.029603. [Google Scholar] [CrossRef]
Joudoi T, Shichiri Y, Kamizono N, Akaike T, Sawa T, Yoshitake J, Yamada N, Iwai S (2013). Nitrated cyclic GMP modulates guard cell signaling in Arabidopsis. Plant Cell 25: 558–571. DOI 10.1105/tpc.112.105049. [Google Scholar] [CrossRef]
Jumali SS, Said IM, Ismail I, Zainal Z (2011). Genes induced by high concentration of salicylic acid in ‘Mitragyna speciosa’. Australian Journal of Crop Science 5: 296–303. [Google Scholar]
Junghans U, Polle A, Düchting P, Weiler E, Kuhlman B et al. (2006). Adaptation to high salinity in poplar involves changes in xylem anatomy and auxin physiology. Plant, Cell & Environment 29: 1519–1531. DOI 10.1111/j.1365-3040.2006.01529.x. [Google Scholar] [CrossRef]
Kai K, Kasa S, Sakamoto M, Aoki N, Watabe G, Yuasa T, Iwaya-Inoue M, Ishibashi Y (2016). Role of reactive oxygen species produced by NADPH oxidase in gibberellin biosynthesis during barley seed germination. Plant Signaling & Behaviour 11: e1180492. DOI 10.1080/15592324.2016.1180492. [Google Scholar] [CrossRef]
Kang GZ, Li GZ, Liu GQ, Xu W, Peng XQ, Wang CY, Zhu YJ, Guo TC (2013). Exogenous salicylic acid enhances wheat drought tolerance by influence on the expression of genes related to ascorbate-glutathione cycle. Biologia Plantarum 57: 718–724. DOI 10.1007/s10535-013-0335-z. [Google Scholar] [CrossRef]
Kangasjärvi J, Jaspers P, Kollist H (2005). Signalling and cell death in ozone-exposed plants. Plant, Cell & Environment 28: 1021–1036. DOI 10.1111/j.1365-3040.2005.01325.x. [Google Scholar] [CrossRef]
Karpets YV, Kolupaev YE, Lugovaya AA, Oboznyi AI (2014). Effect of jasmonic acid on the pro-/antioxidant system of wheat coleoptiles as related to hyperthermia tolerance. Russian Journal of Plant Physiology 61: 339–346. DOI 10.1134/S102144371402006X. [Google Scholar] [CrossRef]
Kawano T (2003). Roles of the reactive oxygen species-generating peroxidase reactions in plant defense and growth induction. Plant Cell Reports 21: 829–837. DOI 10.1007/s00299-003-0591-z. [Google Scholar] [CrossRef]
Khan MI, Asgher M, Khan NA (2014). Alleviation of salt-induced photosynthesis and growth inhibition by salicylic acid involves glycinebetaine and ethylene in mungbean (Vigna radiata L.). Plant Physiology and Biochemistry 80: 67–74. DOI 10.1016/j.plaphy.2014.03.026. [Google Scholar] [CrossRef]
Khan MI, Fatma M, Per TS, Anjum NA, Khan NA (2015). Salicylic acid-induced abiotic stress tolerance and underlying mechanisms in plants. Frontiers in Plant Science 6: 462. DOI 10.3389/fpls.2015.00462. [Google Scholar] [CrossRef]
Khan NA, Khan MI, Ferrante A, Poor P (2017). Ethylene: A key regulatory molecule in plants. Frontiers in Plant Science 8: 1782. DOI 10.3389/fpls.2017.01782. [Google Scholar] [CrossRef]
Khan MI, Syeed S, Nazar R, Anjum NA (2012). An insight into the role of salicylic acid and jasmonic acid in salt stress tolerance. Phytohormones and Abiotic Stress Tolerance in Plants, 277–300. DOI 10.3389/fpls.2015.00462. [Google Scholar] [CrossRef]
Khokon MA, Okuma EI, Hossain MA, Munemasa S, Uraji M et al. (2011). Involvement of extracellular oxidative burst in salicylic acid-induced stomatal closure in Arabidopsis. Plant Cell & Environment 34: 434–443. DOI 10.1111/j.1365-3040.2010.02253.x. [Google Scholar] [CrossRef]
Kim TW, Michniewicz M, Bergmann DC, Wang ZY (2012). Brassinosteroid regulates stomatal development by GSK3-mediated inhibition of a MAPK pathway. Nature 482: 419–422. DOI 10.1038/nature10794. [Google Scholar] [CrossRef]
Klay I, Gouia S, Liu M, Mila I, Khoudi H, Bernadac A, Bouzayen M, Pirrello J (2018). Ethylene Response Factors (ERF) are differentially regulated by different abiotic stress types in tomato plants. Plant Science 274: 137–145. DOI 10.1016/j.plantsci.2018.05.023. [Google Scholar] [CrossRef]
Kliebenstein DJ, Monde RA, Last RL (1998). Superoxide dismutase in Arabidopsis: An eclectic enzyme family with disparate regulation and protein localization. Plant Physiology 118: 637–650. DOI 10.1016/j.plantsci.2018.05.023. [Google Scholar] [CrossRef]
Kollist H, Zandalinas SI, Sengupta S, Nuhkat M, Kangasjärvi J, Mittler R (2019). Rapid responses to abiotic stress: Priming the landscape for the signal transduction network. Trends in Plant Science 24: 25–37. DOI 10.1016/j.tplants.2018.10.003. [Google Scholar] [CrossRef]
Kora D, Bhattacharjee S (2020). The interaction of reactive oxygen species and antioxidants at the metabolic interface in salicylic acid-induced adventitious root formation in mung bean [Vigna radiata (L.) R. Wilczek]. Journal of Plant Physiology 248. DOI 10.1016/j.jplph.2020.153152. [Google Scholar] [CrossRef]
Kumar M, Kesawat MS, Ali A, Lee SC, Gill SS, Kim HU (2019). Integration of abscisic acid signaling with other signaling pathways in plant stress responses and development. Plants 8: 592. DOI 10.3390/plants8120592. [Google Scholar] [CrossRef]
Kwak JM, Mori IC, Pei ZM, Leonhardt N, Torres MA et al. (2003). NADPH oxidase AtrbohD and AtrbohF genes function in ROS-dependent ABA signaling in Arabidopsis. The EMBO Journal 22: 2623–2633. DOI 10.1093/emboj/cdg277. [Google Scholar] [CrossRef]
Lee S, Kim SG, Park CM (2010). Salicylic acid promotes seed germination under high salinity by modulating antioxidant activity in Arabidopsis. New Phytologist 188: 626–637. DOI 10.1111/j.1469-8137.2010.03378.x. [Google Scholar] [CrossRef]
Lee S, Park CM (2010). Modulation of reactive oxygen species by salicylic acid in Arabidopsis seed germination under high salinity. Plant Signaling & Behavior 5: 1534–1536. DOI 10.4161/psb.5.12.13159. [Google Scholar] [CrossRef]
León J, Lawton MA, Raskin I (1995). Hydrogen peroxide stimulates salicylic acid biosynthesis in tobacco. Plant Physiology 108: 1673–1678. DOI 10.1104/pp.108.4.1673. [Google Scholar] [CrossRef]
Li G, Peng X, Wei L, Kang G (2013). Salicylic acid increases the contents of glutathione and ascorbate and temporally regulates the related gene expression in salt-stressed wheat seedlings. Gene 529: 321–325. DOI 10.1016/j.gene.2013.07.093. [Google Scholar] [CrossRef]
Li Z, Yu J, Peng Y, Huang B (2017). Metabolic pathways regulated by abscisic acid, salicylic acid and γ-aminobutyric acid in association with improved drought tolerance in creeping bentgrass (Agrostis stolonifera). Physiologia Plantarum 159: 42–58. DOI 10.1111/ppl.12483. [Google Scholar] [CrossRef]
Lubovská Z, Dobrá J, Storchová H, Wilhelmová N, Vanková R (2014). Cytokinin oxidase/dehydrogenase overexpression modifies antioxidant defense against heat, drought and their combination in Nicotiana tabacum plants. Journal of Plant Physiology 171: 1625–1633. DOI 10.1016/j.jplph.2014.06.021. [Google Scholar] [CrossRef]
Ludwig AA, Saitoh H, Felix G, Freymark G, Miersch O, Wasternack C, Boller T, Jones JD, Romeis T (2005). Ethylene-mediated crosstalk between calcium-dependent protein kinase and MAPK signaling controls stress responses in plants. PNAS 102: 10736–10741. DOI 10.1073/pnas.0502954102. [Google Scholar] [CrossRef]
Lutts S, Kinet JM, Bouharmont J (1996). NaCl-induced senescence in leaves of rice (Oryza sativa L.) cultivars differing in salinity resistance. Annals of Botany 78: 389–398. DOI 10.1006/anbo.1996.0134. [Google Scholar] [CrossRef]
Ma Y, Szostkiewicz I, Korte A, Moes D, Yang Y, Christmann A, Grill E (2009). Regulators of PP2C phosphatase activity function as abscisic acid sensors. Science 324: 1064–1068. DOI 10.1126/science.1172408. [Google Scholar] [CrossRef]
Ma L, Zhang H, Sun L, Jiao Y, Zhang G, Miao C, Hao F (2012). NADPH oxidase AtrbohD and AtrbohF function in ROS-dependent regulation of Na+/K+ homeostasis in Arabidopsis under salt stress. Journal of Experimental Botany 63: 305–317. DOI 10.1093/jxb/err280. [Google Scholar] [CrossRef]
Magome H, Yamaguchi S, Hanada A, Kamiya Y, Oda K (2008). The DDF1 transcriptional activator upregulates expression of a gibberellin-deactivating gene, GA2ox7, under high-salinity stress in Arabidopsis. The Plant Journal 56: 613–626. DOI 10.1111/j.1365-313X.2008.03627.x. [Google Scholar] [CrossRef]
Manar T, Banu G, Fikret Y, Şebnem K, Özlem U, Şebnem E (2013). The effects of JA treatment on the growth and some enzyme activities of eggplant embryos grown in vitro under salt stress conditions. Research Journal of Biotechnology 8: 12. [Google Scholar]
Martin ER, Postiglione AE, Muday GK (2022). Reactive oxygen species function as signalling molecules in controlling plant development and hormonal responses. Current Opinion in Plant Biology 69: 102293. DOI 10.1016/j.pbi.2022.102293. [Google Scholar] [CrossRef]
Masood A, Iqbal N, Khan NA (2012). Role of ethylene in alleviation of cadmium-induced photosynthetic capacity inhibition by sulphur in mustard. Plant, Cell & Environment 35: 524–533. DOI 10.1111/j.1365-3040.2011.02432.x. [Google Scholar] [CrossRef]
Medeiros DB, Barros JAS, Fernie AR, Araujo WL (2020). Eating away at ROS to regulate stomatal opening. Trends in Plant Science 25: 220–223. DOI 10.1016/j.tplants.2019.12.023. [Google Scholar] [CrossRef]
Menke FL, Van Pelt JA, Pieterse CM, Klessig DF (2004). Silencing of the mitogen-activated protein kinase MPK6 compromises disease resistance in Arabidopsis. The Plant Cell 16: 897–907. DOI 10.1105/tpc.015552. [Google Scholar] [CrossRef]
Miller G, Shulaev V, Mittler R (2008). Reactive oxygen signaling and abiotic stress. Physiologia Plantarum 133: 481–489. DOI 10.1111/j.1399-3054.2008.01090.x. [Google Scholar] [CrossRef]
Miller GA, Suzuki N, Ciftci-Yilmaz SU, Mittler RO (2010). Reactive oxygen species homeostasis and signalling during drought and salinity stresses. Plant, Cell & Environment 33: 453–467. DOI 10.1111/j.1365-3040.2009.02041.x. [Google Scholar] [CrossRef]
Minglin L, Yuxiu Z, Tuanyao C (2005). Identification of genes up-regulated in response to Cd exposure in Brassica juncea L. Gene 363: 151–158. DOI 10.1016/j.gene.2005.07.037. [Google Scholar] [CrossRef]
Mittler R (2002). Oxidative stress, antioxidants and stress tolerance. Trends in Plant Science 7: 405–410. DOI 10.1016/S1360-1385(02)02312-9. [Google Scholar] [CrossRef]
Mittler R (2006). Abiotic stress, the field environment and stress combination. Trends in Plant Science 11: 15–19. DOI 10.1016/j.tplants.2005.11.002. [Google Scholar] [CrossRef]
Mittler R (2017). ROS are good. Trends in Plant Science 22: 11–19. DOI 10.1016/j.tplants.2016.08.002. [Google Scholar] [CrossRef]
Mittler R, Blumwald E (2015). The roles of ROS and ABA in systemic acquired acclimation. The Plant Cell 27: 64–70. DOI 10.1105/tpc.114.133090. [Google Scholar] [CrossRef]
Mittler R, Vanderauwera S, Suzuki N, Miller GAD, Tognetti VB, Vandepoele K, Gollery M, Shulaev V, van Breusegem F (2011). ROS signaling: The new wave? Trends in Plant Science 16: 300–309. DOI 10.1016/j.tplants.2011.03.007. [Google Scholar] [CrossRef]
Mittler R, Zandalinas SI, Fichman Y (2022). Reactive oxygen species signalling in plant stress responses. Nature Reviews Molecular Cell Biology 23: 663–679. DOI 10.1038/s41580-022-00499-2. [Google Scholar] [CrossRef]
Miura K, Okamoto H, Okuma E, Shiba H, Kamada H, Hasegawa PM, Murata Y (2013). SIZ1 deficiency causes reduced stomatal aperture and enhanced drought tolerance via controlling salicylic acid-induced accumulation of reactive oxygen species in Arabidopsis. The Plant Journal 73: 91–104. DOI 10.1111/tpj.12014. [Google Scholar] [CrossRef]
Miura K, Tada Y (2014). Regulation of water, salinity, and cold stress responses by salicylic acid. Frontiers in Plant Science 5: 4. DOI 10.3389/fpls.2014.00004. [Google Scholar] [CrossRef]
Mohanta TK, Bashir T, Hashem A, Abd_Allah EF, Khan AL, Al-Harrasi AS (2018). Early events in plant abiotic stress signaling: Interplay between calcium, reactive oxygen species and phytohormones. Journal of Plant Growth Regulation 37: 1033–1049. DOI 10.1007/s00344-018-9833-8. [Google Scholar] [CrossRef]
Müller M, Munné-Bosch S (2015). Ethylene response factors: A key regulatory hub in hormone and stress signaling. Plant Physiology 169: 32–41. DOI 10.1104/pp.15.00677. [Google Scholar] [CrossRef]
Nazar R, Iqbal N, Syeed S, Khan NA (2011). Salicylic acid alleviates decreases in photosynthesis under salt stress by enhancing nitrogen and sulfur assimilation and antioxidant metabolism differentially in two mung bean cultivars. Journal of Plant Physiology 168: 807–815. DOI 10.1016/j.jplph.2010.11.001. [Google Scholar] [CrossRef]
Nazar R, Khan MI, Iqbal N, Masood A, Khan NA (2014). Involvement of ethylene in reversal of salt-inhibited photosynthesis by sulfur in mustard. Physiologia Plantarum 152: 331–344. DOI 10.1111/ppl.12173. [Google Scholar] [CrossRef]
Nazar R, Umar S, Khan NA (2015). Exogenous salicylic acid improves photosynthesis and growth through increase in ascorbate-glutathione metabolism and S assimilation in mustard under salt stress. Plant Signaling & Behavior 10: e1003751. DOI 10.1111/j.1365-3040.2009.02041.x. [Google Scholar] [CrossRef]
Nie WF, Wang MM, Xia XJ, Zhou YH, Shi K et al. (2013). Silencing of tomato RBOH1 and MPK2 abolishes brassinosteroid-induced H2O2 generation and stress tolerance. Plant Cell and Environment 36: 789–803. DOI 10.1111/pce.12014. [Google Scholar] [CrossRef]
Orozco-Cárdenas ML, Ryan CA (2002). Nitric oxide negatively modulates wound signaling in tomato plants. Plant Physiology 130: 487–493. DOI 10.1104/pp.008375. [Google Scholar] [CrossRef]
Palma F, López-Gómez M, Tejera NA, Lluch C (2013). Salicylic acid improves the salinity tolerance of Medicago sativa in symbiosis with Sinorhizobium meliloti by preventing nitrogen fixation inhibition. Plant Science 208: 75–82. DOI 10.1016/j.plantsci.2013.03.015. [Google Scholar] [CrossRef]
Park SY, Fung P, Nishimura N, Jensen DR, Fujii H et al. (2009). Abscisic acid inhibits type 2C protein phosphatases via the PYR/PYL family of START proteins. Science 324: 1068–1071. DOI 10.1126/science.1173041. [Google Scholar] [CrossRef]
Park JE, Park JY, Kim YS, Staswick PE, Jeon J, Yun J, Kim SY, Kim J, Lee YH, Park CM (2007). GH3-mediated auxin homeostasis links growth regulation with stress adaptation response in Arabidopsis. Journal of Biological Chemistry 282: 10036–10046. DOI 10.1074/jbc.M610524200. [Google Scholar] [CrossRef]
Parra-Lobato MC, Fernandez-Garcia N, Olmos E, Alvarez-Tinaut MC, Gómez-Jiménez MC (2009). Methyl jasmonate-induced antioxidant defence in root apoplast from sunflower seedlings. Environmental and Experimental Botany 66: 9–17. DOI 10.1016/j.envexpbot.2009.01.002. [Google Scholar] [CrossRef]
Pei ZM, Murata Y, Benning G, Thomine S, Klüsener B, Allen GJ, Grill E, Schroeder JI (2000). Calcium channels activated by hydrogen peroxide mediate abscisic acid signalling in guard cells. Nature 406: 731–734. DOI 10.1038/35021067. [Google Scholar] [CrossRef]
Peleg Z, Blumwald E (2011). Hormone balance and abiotic stress tolerance in crop plants. Current Opinion in Plant Biology 14: 290–295. DOI 10.1016/j.pbi.2011.02.001. [Google Scholar] [CrossRef]
Pierik R, Tholen D, Poorter H, Visser EJ, Voesenek LA (2006). The Janus face of ethylene: Growth inhibition and stimulation. Trends in Plant Science 11: 176–183. DOI 10.1016/j.tplants.2006.02.006. [Google Scholar] [CrossRef]
Prakash L, Prathapasenan G (1990). NaCl-and gibberellic acid-induced changes in the content of auxin and the activities of cellulase and pectin lyase during leaf growth in rice (Oryza sativa). Annals of Botany 65: 251–257. DOI 10.1093/oxfordjournals.aob.a087931. [Google Scholar] [CrossRef]
Prodhan MY, Munemasa S, Nahar MNEN, Nakamura Y, Murata Y (2018). Guard cell salicylic acid signaling is integrated into abscisic acid signaling via the Ca2+/CPK-dependent pathway. Plant Physiology 178: 441–450. DOI 10.1104/pp.18.00321. [Google Scholar] [CrossRef]
Qiu Z, Guo J, Zhu A, Zhang L, Zhang M (2014). Exogenous jasmonic acid can enhance tolerance of wheat seedlings to salt stress. Ecotoxicology and Environmental Safety 104: 202–208. DOI 10.1016/j.ecoenv.2014.03.014 [Google Scholar] [CrossRef]
Raeymaekers T, Potters G, Asard H, Guisez Y, Horemans N (2003). Copper-mediated oxidative burst in Nicotiana tabacum L. cv. Bright Yellow 2 cell suspension cultures. Protoplasma 221: 93–100. DOI 10.1007/s00709-002-0063-2 [Google Scholar] [CrossRef]
Raja V, Majeed U, Kang H, Andrabi KI, John R (2017). Abiotic stress: Interplay between ROS, hormones and MAPKs. Environmental and Experimental Botany 137: 142–157. DOI 10.1016/j.envexpbot.2017.02.010. [Google Scholar] [CrossRef]
Rajjou L, Belghazi M, Huguet R, Robin C, Moreau A, Job C, Job D (2006). Proteomic investigation of the effect of salicylic acid on Arabidopsis seed germination and establishment of early defense mechanisms. Plant Physiology 141: 910–923. DOI 10.1104/pp.106.082057. [Google Scholar] [CrossRef]
Reid MS (1995). Ethylene in plant growth, development, and senescence. In: Davis PJ, eds., Plant Hormones, pp. 486–508. Springer, Dordrecht. [Google Scholar]
Sah SK, Reddy KR, Li J (2016). Abscisic acid and abiotic stress tolerance in crop plants. Frontiers in Plant Science 7: 571. DOI 10.3389/fpls.2016.00571. [Google Scholar] [CrossRef]
Sakamoto M, Munemura I, Tomita R, Kobayashi K (2008). Involvement of hydrogen peroxide in leaf abscission signaling, revealed by analysis with an in vitro abscission system in Capsicum plants. The Plant Journal 56: 13–27. DOI 10.1111/j.1365-313X.2008.03577.x. [Google Scholar] [CrossRef]
Salzman RA, Brady JA, Finlayson SA, Buchanan CD, Summer EJ, et al. (2005). Transcriptional profiling of sorghum induced by methyl jasmonate, salicylic acid, and aminocyclopropane carboxylic acid reveals cooperative regulation and novel gene responses. Plant Physiology 138: 352–368. DOI 10.1104/pp.104.058206. [Google Scholar] [CrossRef]
Santelia D, Henrichs S, Vincenzetti V, Sauer M, Bigler L et al. (2008). Flavonoids redirect PIN-mediated polar auxin fluxes during root gravitropic responses. Journal of Biological Chemistry 283: 31218–31226. DOI 10.1074/jbc.M710122200. [Google Scholar] [CrossRef]
Santiago J, Dupeux F, Round A, Antoni R, Park SY et al. (2009a). The abscisic acid receptor PYR1 in complex with abscisic acid. Nature 462: 665–668. DOI 10.1038/nature08591. [Google Scholar] [CrossRef]
Santiago J, Rodrigues A, Saez A, Rubio S, Antoni R et al. (2009b). Modulation of drought resistance by the abscisic acid receptor PYL5 through inhibition of clade A PP2Cs. The Plant Journal 60: 575–588. DOI 10.1111/j.1365-313X.2009.03981.x. [Google Scholar] [CrossRef]
Schoneich C (2000). Mechanisms of metal-catalyzed oxidation of histidine to 2-oxo-histidine in peptides and proteins. Journal of Pharmaceutical and Biomedical Analysis 21: 1093–1097. DOI 10.1016/S0731-7085(99)00182-X. [Google Scholar] [CrossRef]
Sewelam N, Kazan K, Thomas-Hall SR, Kidd BN, Manners JM, Schenk PM (2013). Ethylene response factor 6 is a regulator of reactive oxygen species signaling in Arabidopsis. PLoS One 8: e70289. DOI 10.1371/journal.pone.0070289. [Google Scholar] [CrossRef]
Shan C, Mei Z, Duan J, Chen H, Feng H, Cai W (2014). OsGA2ox5, a gibberellin metabolism enzyme, is involved in plant growth, the root gravity response and salt stress. PLoS One 9: e87110. DOI 10.1371/journal.pone.0087110. [Google Scholar] [CrossRef]
Sharma P, Jha AB, Dubey RS, Pessarakli M (2012). Reactive oxygen species, oxidative damage, and antioxidative defense mechanism in plants under stressful conditions. Journal of Botany 2012: 1–26. DOI 10.1155/2012/217037. [Google Scholar] [CrossRef]
Shi H, Wang X, Ye T, Chen F, Deng J, et al. (2014). The Cysteine2/Histidine2-type transcription factor ZINC FINGER OF ARABIDOPSIS THALIANA6 modulates biotic and abiotic stress responses by activating salicylic acid-related genes and C-REPEAT-BINDING FACTOR genes in Arabidopsis. Plant Physiology 165: 1367–1379. DOI 10.1104/pp.114.242404. [Google Scholar] [CrossRef]
Sies H (2021). Oxidative eustress: On constant alert for redox homeostasis. Redox Biology 41: 101867. DOI 10.1016/j.redox.2021.101867. [Google Scholar] [CrossRef]
Skalak J, Nicolas KL, Vankova R, Hejatko J (2021). Signal integration in plant abiotic stress responses via multistep phosphorelay signaling. Frontiers in Plant Science 12: 644823. DOI 10.3389/fpls.2021.644823. [Google Scholar] [CrossRef]
Soares AM, Souza TF, Jacinto T, Machado OL (2010). Effect of methyl jasmonate on antioxidative enzyme activities and on the contents of ROS and H2O2 in Ricinus communis leaves. Brazilian Journal of Plant Physiology 22: 151–158. DOI 10.1590/S1677-04202010000300001. [Google Scholar] [CrossRef]
Souri Z, Karimi N, Farooq MA, Sandalio LM (2020). Nitric oxide improves tolerance to arsenic stress in Isatis cappadocica desv. Shoots by enhancing antioxidant defenses. Chemosphere 239: 124523. DOI 10.1016/j.chemosphere.2019.124523. [Google Scholar] [CrossRef]
Steffens B, Steffen-Heins A, Sauter M (2013). Reactive oxygen species mediate growth and death in submerged plants. Frontiers in Plant Science 4: 179. DOI 10.3389/fpls.2013.00179. [Google Scholar] [CrossRef]
Suarez-Rodriguez MC, Adams-Phillips L, Liu Y, Wang H, Su SH, Jester PJ, Zhang S, Bent AF, Krysan PJ (2007). MEKK1 is required for flg22-induced MPK4 activation in Arabidopsis plants. Plant Physiology 143: 661–669. DOI 10.1104/pp.106.091389. [Google Scholar] [CrossRef]
Sun Y, Fan XY, Cao DM, Tang W, He K et al. (2010). Integration of brassinosteroid signal transduction with the transcription network for plant growth regulation in Arabidopsis. Developmental Cell 19: 765–777. DOI 10.1016/j.devcel.2010.10.010. [Google Scholar] [CrossRef]
Suzuki N, Bassil E, Hamilton JS (2016). ABA is required for plant acclimation to a combination of salt and heat stress. PLoS One 11: e0147625. DOI 10.1371/journal.pone.0147625. [Google Scholar] [CrossRef]
Suzuki N, Katano K (2018). Coordination between ROS regulatory systems and other pathways under heat stress and pathogen attack. Front of Plant Science 9: 490. DOI 10.3389/fpls.2018.00490. [Google Scholar] [CrossRef]
Takahashi F, Yoshida R, Ichimura K, Mizoguchi T, Seo S et al. (2007). The mitogen-activated protein kinase cascade MKK3-MPK6 is an important part of the jasmonate signal transduction pathway in Arabidopsis. The Plant Cell 19: 805–818. DOI 10.1105/tpc.106.046581. [Google Scholar] [CrossRef]
Thao NP, Khan MI, Thu NB, Hoang XL, Asgher M, Khan NA, Tran LSP (2015). Role of ethylene and its cross talk with other signaling molecules in plant responses to heavy metal stress. Plant Physiology 169: 73–84. DOI 10.1104/pp.15.00663. [Google Scholar] [CrossRef]
Thevenet D, Pastor V, Baccelli I, Balmer A, Vallat A et al. (2017). The priming molecule β-aminobutyric acid is naturally present in plants and is induced by stress. New Phytologist 213: 552–559. DOI 10.1111/nph.14298. [Google Scholar] [CrossRef]
Thompson JE, Froese CD, Madey E, Smith MD, Hong Y (1998). Lipid metabolism during plant senescence. Progress in Lipid Research 37: 119–141. DOI 10.1016/S0163-7827(98)00006-X. [Google Scholar] [CrossRef]
Tognetti VB, Mühlenbock PE, Van Breusegem F (2012). Stress homeostasis-the redox and auxin perspective. Plant, Cell & Environment 35: 321–333. DOI 10.1111/j.1365-3040.2011.02324.x [Google Scholar] [CrossRef]
Tognetti VB, van Aken O, Morreel K, Vandenbroucke K, van de Cotte B et al. (2010). Perturbation in indole-3-butyric acid homeostasis by the UDP-glucosyltransferase UTG74E2 modulates Arabidopsis architecture and water stress tolerance. The Plant Cell 22: 2660–2679. DOI 10.1105/tpc.109.071316. [Google Scholar] [CrossRef]
Torres MA, Dangl JL, Jones JD (2002). Arabidopsis gp91phox homologues AtrbohD and AtrbohF are required for accumulation of reactive oxygen intermediates in the plant defense response. PNAS 99: 517–522. DOI 10.1073/pnas.012452499. [Google Scholar] [CrossRef]
Uchida K, Kawakishi S (1993). 2-Oxo-histidine as a novel biological marker for oxidatively modified proteins. FEBS Letters 332: 208–210. DOI 10.1016/0014-5793(93)80632-5. [Google Scholar] [CrossRef]
van Verk MC, Bol JF, Linthorst HJ (2011). WRKY transcription factors involved in activation of SA biosynthesis genes. BMC Plant Biology 11: 89. DOI 10.1186/1471-2229-11-89. [Google Scholar] [CrossRef]
Verma V, Ravindran P, Kumar PP (2016). Plant hormone-mediated regulation of stress responses. BMC Plant Biology 16: 1. DOI 10.1186/s12870-016-0771-y. [Google Scholar] [CrossRef]
von Sonntag C (1987). The Chemical Basis of Radiation Biology, pp. 221–294. London: Taylor & Francis. [Google Scholar]
Walia H, Wilson C, Condamine P, Liu X, Ismail AM, Close TJ (2007). Large-scale expression profiling and physiological characterization of jasmonic acid-mediated adaptation of barley to salinity stress. Plant, Cell & Environment 30: 410–421. DOI 10.1111/j.1365-3040.2006.01628.x. [Google Scholar] [CrossRef]
Wang J, Song L, Gong X, Xu J, Li M (2020). Functions of jasmonic acid in plant regulation and response to abiotic stress. International Journal of Molecular Sciences 21: 1446. DOI 10.3390/ijms21041446. [Google Scholar] [CrossRef]
Wang C, Yang A, Yin H, Zhang J (2008). Influence of water stress on endogenous hormone contents and cell damage of maize seedlings. Journal of Integrative Plant Biology 50: 427–434. DOI 10.1111/j.1774-7909.2008.00638.x. [Google Scholar] [CrossRef]
Wani SH, Kumar V, Shriram V, Sah SK (2016). Phytohormones and their metabolic engineering for abiotic stress tolerance in crop plants. The Crop Journal 4: 162–176. DOI 10.1016/j.cj.2016.01.010. [Google Scholar] [CrossRef]
Wasternack C (2014). Action of jasmonates in plant stress responses and development—Applied aspects. Biotechnology Advances 32: 31–39. DOI 10.1016/j.biotechadv.2013.09.009. [Google Scholar] [CrossRef]
Wolf S, Hématy K, Höfte H (2012). Growth control and cell wall signaling in plants. Annual Review of Plant Biology 63: 381–407. DOI 10.1146/annurev-arplant-042811-105449. [Google Scholar] [CrossRef]
Wu H, Wu X, Li Z, Duan L, Zhang M (2012). Physiological evaluation of drought stress tolerance and recovery in cauliflower (Brassica oleracea L.) seedlings treated with methyl jasmonate and coronatine. Journal of Plant Growth Regulation 31: 113–123. DOI 10.1007/s00344-011-9224-x. [Google Scholar] [CrossRef]
Wu L, Zhang Z, Zhang H, Wang XC, Huang R (2008). Transcriptional modulation of ethylene response factor protein JERF3 in the oxidative stress response enhances tolerance of tobacco seedlings to salt, drought, and freezing. Plant Physiology 148: 1953–1963. DOI 10.1104/pp.108.126813. [Google Scholar] [CrossRef]
Xia XJ, Gao CJ, Song LX, Zhou YH et al. (2014). Role of H2O2 dynamics in brassinosteroid-induced stomatal closure and opening in Solanum lycopersicum. Plant, Cell & Environment 37: 2036–2050. DOI 10.1111/pce.12275. [Google Scholar] [CrossRef]
Xia XJ, Wang YJ, Zhou YH, Tao Y, Mao WH, Shi K, Asami T, Chen Z, Yu JQ (2009). Reactive oxygen species are involved in brassinosteroid-induced stress tolerance in cucumber. Plant Physiology 150: 801–814. DOI 10.1104/pp.109.138230. [Google Scholar] [CrossRef]
Xia XJ, Zhou YH, Shi K, Zhou J, Foyer CH, Yu JQ (2015). Inter-play between reactive oxygen species and hormones in the control of plant development and stress tolerance. Journal of Experimental Botany 66: 2839–2856. DOI 10.1093/jxb/erv089. [Google Scholar] [CrossRef]
Xiong L, Schumaker KS, Zhu JK (2002). Cell signaling during cold, drought, and salt stress. The Plant Cell 14: S165–S183. DOI 10.1105/tpc.000596. [Google Scholar] [CrossRef]
Xu X, Barry DC, Settleman J, Schwartz MA, Bokoch GM (1994). Differing structural requirements for GTPase-activating protein responsiveness and NADPH oxidase activation by Rac. Journal of Biological Chemistry 269: 23569–23574. DOI 10.1016/S0021-9258(17)31553-3. [Google Scholar] [CrossRef]
Yan C, Yan Z, Wang Y, Yan X, Han Y (2014). Tudor-SN a component of stress granules, regulates growth under salt stress by modulating GA20ox3 mRNA levels in Arabidopsis. Journal of Experimental Botany 65: 5933–5944. DOI 10.1093/jxb/eru334. [Google Scholar] [CrossRef]
Yang W, Zhu C, Ma X, Li G, Gan L, Ng D, Xia K (2013). Hydrogen peroxide is a second messenger in the salicylic acid-triggered adventitious rooting process in mung bean seedlings. PLoS One 8: e84580. DOI 10.1371/journal.pone.0084580. [Google Scholar] [CrossRef]
Yoshida T, Obata T, Feil R, Lunn JE, Fujita Y, Yamaguchi-Shinozaki K, Fernie AR (2019). The role of abscisic acid signaling in maintaining the metabolic balance required for Arabidopsis growth under non-stress conditions. Plant Cell 31: 84–105. DOI 10.1105/tpc.18.00766. [Google Scholar] [CrossRef]
Youm JW, Jeon JH, Choi D, Yi SY, Joung H, Kim HS (2008). Ectopic expression of pepper CaPF1 in potato enhances multiple stresses tolerance and delays initiation of in vitro tuberization. Planta 228: 701–708. DOI 10.1007/s00425-008-0782-5. [Google Scholar] [CrossRef]
Zandalinas SI, Balfagόn D, Arbona V, Gόmez-Cadenas A, Inupakutika MA, Mittler R (2016). ABA is required for the accumulation of APX1 and MBF1c during a combination of water deficit and heat stress. Journal of Experimental Botany 67: 5381–5390. DOI 10.1093/jxb/erw299. [Google Scholar] [CrossRef]
Zandalinas SI, Fichman Y, Devireddy AR, Sengupta S, Azad RK, Mittler R (2020a). Systemic signaling during abiotic stress combination in plants. PNAS 117: 13810–13820. DOI 10.1073/pnas.2005077117. [Google Scholar] [CrossRef]
Zandalinas SI, Fritschi FB, Mittler R (2020b). Signal transduction networks during stress combination. Journal of Experimental Botany 71: 1734–1741. DOI 10.1093/jxb/erz486. [Google Scholar] [CrossRef]
Zandalinas SI, Mittler R, Balfagon D, Arbona V, Gomez-Cadenas A (2018). Plant adaptations to the combination of drought and high temperatures. Physiologia Plantarum 162: 2–12. DOI 10.1111/ppl.12540. [Google Scholar] [CrossRef]
Zengin F (2014). Exogenous treatment with salicylic acid alleviating copper toxicity in bean seedlings. Proceedings of the National Academy of Sciences, India Section B: Biological Sciences 84: 749–755. DOI 10.1007/s40011-013-0285-4. [Google Scholar] [CrossRef]
Zhang A, Jiang M, Zhang J, Tan M, Hu X (2006). Mitogen-activated protein kinase is involved in abscisic acid-induced antioxidant defense and acts downstream of reactive oxygen species production in leaves of maize plants. Plant Physiology 141: 475–487. DOI 10.1104/pp.105.075416. [Google Scholar] [CrossRef]
Zhang Z, Wang J, Zhang R, Huang R (2012). The ethylene response factor AtERF98 enhances tolerance to salt through the transcriptional activation of ascorbic acid synthesis in Arabidopsis. The Plant Journal 71: 273–287. DOI 10.1111/j.1365-313X.2012.04996.x. [Google Scholar] [CrossRef]
Zhang Y, Xu S, Ding P, Wang D, Cheng YT, He J et al. (2010a). Control of salicylic acid synthesis and systemic acquired resistance by two members of a plant-specific family of transcription factors. PNAS 107: 18220–18225. DOI 10.1073/pnas.1005225107. [Google Scholar] [CrossRef]
Zhang X, Zhang L, Dong F, Gao J, Galbraith DW, Song CP (2001). Hydrogen peroxide is involved in abscisic acid-induced stomatal closure in Vicia faba. Plant Physiology 126: 1438–1448. DOI 10.1104/pp.126.4.1438. [Google Scholar] [CrossRef]
Zhang A, Zhang J, Ye N, Cao J, Tan M, Zhang J, Jiang M (2010b). ZmMPK5 is required for the NADPH oxidase-mediated self-propagation of apoplastic H2O2 in brassinosteroid-induced antioxidant defence in leaves of maize. Journal of Experimental Botany 61: 4399–4411. DOI 10.1093/jxb/erq243. [Google Scholar] [CrossRef]
Zhang Y, Zhu H, Zhang Q, Li M, Yan M et al. (2009). Phospholipase Dα1 and phosphatidic acid regulate NADPH oxidase activity and production of reactive oxygen species in ABA-mediated stomatal closure in Arabidopsis. The Plant Cell 21: 2357–2377. DOI 10.1105/tpc.108.062992. [Google Scholar] [CrossRef]
Zhou J, Wang J, Li X, Xia XJ, Zhou YH et al. (2014). H2O2 mediates the crosstalk of brassinosteroid and abscisic acid in tomato responses to heat and oxidative stresses. Journal of Experimental Botany 65: 4371–4383. DOI 10.1093/jxb/eru217. [Google Scholar] [CrossRef]
Zhu X, Feng Y, Liang G, Liu N, Zhu JK (2013). Aequorin-based luminescence imaging reveals stimulus-and tissue-specific Ca2+ dynamics in Arabidopsis plants. Molecular Plant 6: 444–455. DOI 10.1093/mp/sst013. [Google Scholar] [CrossRef]
Cite This Article
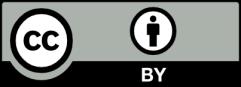
This work is licensed under a Creative Commons Attribution 4.0 International License , which permits unrestricted use, distribution, and reproduction in any medium, provided the original work is properly cited.