Open Access
REVIEW
Translocation and transformation of engineered nanomaterials in plant cells and their effect on metabolism
1 Beijing Key Laboratory of Farmland Soil Pollution Prevention and Remediation, College of Resources and Environmental Sciences, China Agricultural University, Beijing, 100193, China
2 China Agricultural University Professor Workstation of Yuhuangmiao Town, Jinan, 251600, China
3 China Agricultural University Professor Workstation of Sunji Town, Jinan, 251600, China
4 Jiaer Chen Academician Workstation, Jinan, 251600, China
* Corresponding Author: YUKUI RUI. Email:
(This article belongs to the Special Issue: Advances in Plant Cell Biology Research)
BIOCELL 2023, 47(3), 493-502. https://doi.org/10.32604/biocell.2023.025740
Received 10 August 2022; Accepted 09 September 2022; Issue published 03 January 2023
Abstract
As the climate worsens and the demand for food grows, so does the interest in nanoagriculture. The interaction between plants and nanomaterials (NMs) has been extensively and intensively examined. However, stopping at the outcome of a phenomenon is often insufficient. Therefore, we introduce three important processes of nanoparticle-plant interactions: translocation, transformation, and plant metabolism. During the migration of nanoparticles, size and surface electrical properties are the main determining factors. Additionally, the interaction of nanoparticles with cell membranes is another key aspect of research. The transformation of nanoparticles in plants is mainly due to redox substances. The way that nanoparticles affect plant metabolism may be able to shed light on the interaction of nanoparticles with plants. This review adds to the existing knowledge on the design of nanoagrochemicals and summarizes the mechanism of interaction of NMs with plants. In this way, NMs can be used for their beneficial effects and thus contribute to the maintenance of food security and sustainable development.Keywords
As the population continues to grow, the demand for food gradually increases. By 2050, the demand for food will rise by 70% (Bindraban et al., 2018). In the face of food needs and security, nanomaterials (NMs) emerge as a promising solution. Traditional agrochemicals cause some environmental pollution, and although they are of great help to agricultural production, the cost is also unaffordable for people. Climate degradation is also urging an accelerated exploration of nanotechnology applications in agricultural environments. Currently, the NMs are used in a number of environmental applications, including the reduction of air pollution (Lou et al., 2022; Zhao et al., 2022b), mitigation of heavy metal stress (Zhou et al., 2021b), and water purification (Chong et al., 2010; Jjagwe et al., 2021). The more thorough investigation has also inadvertently assisted in the understanding of NMs’ effects on plant physiology (Faizan et al., 2021; Iannone et al., 2014; Juarez-Maldonado, 2022; Juárez-Maldonado, 2022). It has been demonstrated that NMs can encourage plant development and enhance the condition of plants’ growth (Adeel et al., 2021; Farooq et al., 2021; Li et al., 2021; Pang et al., 2021; Shakoor et al., 2022; Wang et al., 2020). However, NMs are like two sides of the same coin, and some studies have pointed out that NMs may have adverse effects on plants under certain conditions (Bai et al., 2021; Guo et al., 2022; Wang et al., 2019). Excessive amounts of NMs can be toxic and hinder the growth and development of soil microorganisms and plants (Chen et al., 2022; Dev et al., 2018; Li et al., 2022; Sardoiwala et al., 2018; Yusefi-Tanha et al., 2022; Zhao et al., 2022a). The accumulation of NMs in the environment poses a concern to the human body since they can enter through a number of different channels (Moore, 2006; Sharifi et al., 2012; Zhang et al., 2018).
Understanding the biological impacts of NMs on plants requires an understanding of their transport, change, and impact on plant metabolism (Lin et al., 2009; Schymura et al., 2017). The application of NMs includes foliar, root application, and seed germination (Wang et al., 2022d; Yue et al., 2022; Zhao et al., 2020). The NMs migrate into the plant, which has been explored in depth in a previous review (Dietz and Herth, 2011; Lv et al., 2019; Siddiqi and Husen, 2017; Singh et al., 2018; Usman et al., 2020; Verma et al., 2019). We are especially interested in the migration of NMs at the cellular level (Gao et al., 2019; Hua et al., 2021; Qu et al., 2022), which has gotten less attention. The size of the nanoparticles (NPs), which are the main barriers to NM entrance into plant cells, has the biggest impact. The barriers to entry of NPs into plant cells mainly consist of cell walls and membrane structures. The charge carried by NPs and cell wall and membrane structures is also one of the factors worth considering (Hu et al., 2020). We will describe it in detail below. The migration is accompanied by the transformation of NPs, and the transformation also facilitates the migration of elemental species to some extent. For example, in ZnO NPs as nanopesticides, the Zn2+ ion exhibits the antibacterial effect. Reactive oxygen species (ROS) that have a sterilizing effect, are also produced by ZnO NPs (Khan et al., 2016; Molnár et al., 2020; Wagner et al., 2016). The entry of NPs into plants also affects plant metabolism indirectly through phytohormones and light and efficiency, which will be described in detail here.
We searched the web of science using the subject terms (1) “plants” and “nanomaterials” and “transfer”; (2) “plants” and “nanomaterials” and “transformation”; (3) “plants” and “nanomaterials” and “metabolism”. A total of 70 reviews were screened, and keyword co-occurrence analysis was performed on these 70 reviews (Fig. 1). In the co-occurrence illustration (Fig. 1), we could draw some conclusions from the current reviews: (1) There are only a few reviews on the effects of the migration transformation process of nanoparticles; (2) Applications for nano-agriculture, such as nano-fertilizers and nano-sensors, are increasingly becoming research topics of interest; (3) Engineered nanomaterials have a large body of research and are a major research target for studying plant-nanomaterial interactions.
Figure 1: An illustration of co-occurrence analysis of a review on nanomaterial migration, transformation, and impact on plant metabolism.
This review introduces the migration, transformation, and influence of NPs on plant metabolism (Fig. 2). The mechanisms of these processes and the factors affecting the migration and transformation of NPs were explored, and challenges and future research directions are highlighted. The novelty of this paper lies in the overview of the important activities faced in the internalization of nanoparticles and the presentation of the process from the outside to the inside of the plant system. This review thus sheds light on the current research on NPs at the plant cellular level.
Figure 2: Illustration of the variables that affect plant metabolism, nanoparticle translocation, and nanoparticle transformation.
The Translocation of Nanomaterials in Plant
NMs are structurally prevented from entering the plant cell walls and membranes. NPs also interact with the elements of the cell wall and membrane. For the design and application of NPs, it is crucial to investigate the challenges and influencing factors encountered during the migration of NPs (Fig. 3). We will next discuss the structure of plant cells from the outside to the inside, in terms of the cell wall dimensions, the interaction of NMs with the cell wall and the interaction of NMs with the membrane structure in turn.
Figure 3: Illustration of possible barriers to the entry of NPs into plant cells.
The pore size of plant cell wall
The cell wall is a more solid, durable, and slightly more flexible structure than the cell membrane. Cellulose, hemicellulose, and lignin make up the majority of the cell wall (Zeng et al., 2017). Plant cells must face the engineered nanomaterials before they may enter the cell. Plant cell walls are made up of a cross-linked network of pectins and proteins (Shomer et al., 2003). Also, the cross-linked network determines the size of the wall pores of dicotyledonous cells (Fleischer et al., 1999). The size of the cell wall prevents NMs from migrating further (Wu and Li, 2022). Therefore, it is crucial to comprehend the size of the cell wall pores (Carpita et al., 1979; Lew et al., 2018). For different plants, the porosity tends to differ (Chesson et al., 1997; Fujino and Itoh, 1998). The radius of the wheat (Triticum aestivum L.) cell wall pores is 1.5–3 nm (Chesson et al., 1997). The pore size of maize (Z. mays L.) stover cell walls is between 10 and 1000 nm, although it varies depending on where it is located (Chundawat et al., 2011). In one experiment, 11 nm NPs were discovered on the leaves of the maize (Hu et al., 2020). The epidermal cells of the pea (Pisum sativum) feature pores that are typically 5.5 ± 1.4 nm in size for their elongating cell wall and 13.4 ± 4.3 nm for their non-elongating cell wall (Fujino and Itoh, 1998). The STEM examination of NPs isolated from leaves revealed a 10 nm-diameter of Zr/CeOx NPs (Schwabe et al., 2015). When the particle size was more than 20 nm, no root-to-shoot translocation was seen. The same outcomes of a second experiment demonstrated that 18 nm size Fe2O3 NPs could not be transported from roots to shoots of maize (Li et al., 2016).
In general, NPs smaller than 13 nm in size are able to enter plant cells directly through cell wall pores, while those with larger sizes, such as 40–60 nm, can enter plant cells through intercellular filaments.
The interaction between nanomaterials and the cell wall
The ability of NMs to pass through the cell wall may be influenced by elements other than the size of the cell wall pores. There may be more complex interactions between the NMs and the cell wall.
Transmission electron microscopy studies suggest that the root cell wall of Brassica (Brassica napus) treated with ZnO NPs at a concentration of 25 mg/L was bound to Zn2+ (Molnár et al., 2020). This demonstrates the capacity of ZnO NPs to modify cell membranes. Because plant cell walls are active, they can easily redesign themselves in response to environmental changes (Houston et al., 2016). After being exposed to Y2O3 NPs, the form and composition of cell wall of tobacco (Nicotiana tabacum L.) were also altered. The cell wall thickness of tobacco increased significantly by 7–12 times, the pectin content increased by 58%, and the hemicellulose content decreased by 29% (Chen et al., 2021). In the presence of ROS, Ag+ produced by Ag NPs can bind to hydroxy cellulose structures, breaking hydrogen bonds to promote changes in cell wall structure (Pinheiro et al., 2021). Pea (Pisum sativum L.) growth exposed to TiO2 NPs exhibited altered polysaccharide content of the cell wall (Fan et al., 2014). Similar to this, Fourier transform infrared spectroscopy studies revealed that TiO2 NPs altered the composition of the leaf cell walls of tomatoes (Solanum lycopersicum L.). The alterations in the amounts of xyloglucan and hypergalacturonic acid were validated by microarray polymer analysis (Line et al., 2021). Another intriguing study looked at nZVI’s potential to encourage root growth in Arabidopsis (Arabidopsis thaliana). The concentration of 0.5 g/L nZVI increased root elongation by 150%–200% via inducing OH radical-induced cell wall loosening and the beginning of H2O2 release (Kim et al., 2014).
Electrical characteristics based on pore size are the second most crucial component to take into account. In a previous study, maize leaves were exposed to positively and negatively charged NPs on their surface, but their size was larger than 11 nm, which possibly limited their access to the interior of the plant (Hu et al., 2020). The electrical potential of the cell wall is another factor that must be considered. The electrical charge in the cell wall is typically between −50 and −110 mV (Shomer et al., 2003). The NPs with a charge opposite to that of the cell wall may be retained by the cell wall (Bao et al., 2016; Juarez-Maldonado et al., 2019). Negatively charged Au NPs were found in the roots of Arabidopsis (Arabidopsis thaliana), and positively charged Au NPs were not detected in the roots cell (Avellan et al., 2017). Negative polarity Se NPs were more abundant in the Bok Choy (Brassica chinensis L.) shoots (Wang et al., 2022b). This also shows that negatively charged cell walls may play a role in the retention of positively charged NPs in roots, thus giving us ideas for the creation of nanopesticides or nanofertilizers in the future. Positively charged NPs, on the other hand, appear to be better at moving within plants, according to some studies. Indeed, the positively charged ZnO NPs allowed their adsorption on the leaf surface and cell walls and were more uniformly distributed within the leaf than the negatively charged ones (Zhu et al., 2021).
NMs have also been shown to alter the composition of cell walls. As mentioned previously, Y2O3 NPs can alter the pectin and hemicellulose content of plant cell walls (Chen et al., 2021). CuO NPs also reduced the amount of cellulose, hemicellulose, pectin, and other monosaccharides in the tomato (Solanum lycopersicum L.) root cell wall (Jia et al., 2022). Molnár et al. (2020) discovered that NP concentrations must exceed a specific threshold to modify the structure of the cell wall.
The role of cell wall potential in the uptake of NPs by plants and whether or not interactions between NPs and plant cell walls are connected with different cell types are both poorly understood. As a result, we must consider factors including the electrical gradient across the cell wall, NP size, interactions with cell wall components, and NP concentration that also influence NP movement inside the plant.
The interaction between nanomaterials and the membrane
NPs must overcome the membrane barrier after passing through the cell wall (Wu and Li, 2022). The plasma membrane, a border membrane that encloses the contents of the cell, is normally 5–10 nm thick. Some of the methods of cellular internalization include endocytosis, penetration through transporters or channels in the cell membrane, and lipid exchange envelopes (Lv et al., 2021; Perozo et al., 2002; Schwab et al., 2016; Sosan et al., 2016; Wong et al., 2016; Wu et al., 2017).
Similar to plant cell walls, the membrane potential plays a crucial role in controlling the flow of NPs through the membrane (Wu and Li, 2022). The plasma membrane potential is greater than that of the cell wall, at roughly −120 mV (Wu et al., 2013). High-zeta potential NPs are capable of entering protoplasts (Lew et al., 2018). However, it has been found that Au NPs crossing the plasma membrane are not affected by their electrical properties (Milewska-Hendel et al., 2019). The usage of 5 nm Au NPs, which are within the range of passing size for the channels on the plasma membrane, may be the cause of this. NPs are also able to infiltrate organelles other than protoplasts by traversing organelle membranes (Liu et al., 2021; Milewska-Hendel et al., 2019; Wu et al., 2017; Zhou et al., 2021a). The interaction of NPs with membranes has received little attention, although it might be one of the most intriguing areas for future study.
The Transformation of Nanomaterials in Plant
In the plant, the NPs may undergo transformation (Table 1), producing a number of elemental species (Lv et al., 2019). This process is mainly due to (1) root secretions or (2) the interaction of biofilms around the root system, and (3) redox species in the plant.
After being adsorbed on the surface of plant roots, NPs undergo oxidative solubilization to pass the cell membrane, and after internalization, NPs can move within and across plant tissues. X-ray spectro-microscopy revealed that Ag NPs were oxidized within the root tissue of Lolium multiflorum (Yin et al., 2011). Ag NPs are oxidized directly inside the root tissue or are oxidized, and the Ag+ is absorbed by the roots. Alfalfa sprouts can absorb Ag+ from the culture medium and reduce it to Ag NPs in their bodies. Alfalfa plant tissues contain the accumulated Ag atoms that undergo processes related to nucleation and NPs production (Gardea-Torresdey et al., 2003). Similarly, Au underwent a reduction reaction in alfalfa plants to produce Au NPs (Gardea-Torresdey et al., 2002). At that time, these findings were very interesting and may provide some new inspiration for the development of green synthetic NPs (Jiang et al., 2022). The metal oxides can vary in a way that affects plant accumulation and species creation. When treated with ZnO NPs, the roots and shoots of the maize plant absorb Zn predominantly in ionic form. The biotransformation of ZnO NPs into ZnO phosphate in the plant limits their long-distance transport since there is relatively little upward migration of Zn in the shoots (Lv et al., 2015). CuO and Cu(I)-sulfur complexes are the main forms of bioaccumulated copper in wheat (Triticum aestivum L.), whereas the phosphate form is the main form of bioaccumulated Zn (Dimkpa et al., 2012). This is principally brought upon by an increase in the solubilization of ZnO NP between the roots as well as plant uptake and translocation of Zn ions (Lv et al., 2015). Zn was discovered in soybean (Glycine max) root tissue as Zn-nitrate and Zn-acetate (Lopez-Moreno et al., 2010). In subsequent experiments, Zn-citrate was detected by the mu-XANES technique (Hernandez-Viezcas et al., 2013). Similarly, different forms of Zn were also found in other plants (Hernandez-Viezcas et al., 2011; Wang et al., 2013). Despite the presence of numerous Zn species in the plants under study, it is clear that Zn uptake, transport, and accumulation in plants primarily take place in the form of Zn2+ produced by ZnO NPs (Lv et al., 2019). The CuO NPs and ZnO NPs are the NPs with more applications in agriculture (Wang et al., 2022c). Zn uptake, transport, and accumulation in plants happen largely in the form of Zn2+ released by ZnO NPs, although many Zn species were found in the plants under study (Dimkpa et al., 2012). In maize, CuO NPs move from the branches back to the roots through the bast, where they can be converted to Cu(I) (Wang et al., 2012). While a portion of the dissolved Cu(II) was also reduced to Cu2O, it was mostly coupled with ligands like cysteine, citrate, and phosphate. Additionally, rice roots were found to transfer 40 nm CuO NPs to the shoots (Peng et al., 2015). In cucumbers exposed to CeO2 NPs, Ce occurs as CeO2 and CePO4 in the roots and as CeO2 and Ce-carboxylic acid in the branches, according to XANES spectra (Zhang et al., 2012). The primary causes could be (1) the function of ascorbic acid as a reducing agent and (2) the organic acids-mediated dissolution of CeO2 NPs. Other tests have shown similar outcomes (Cui et al., 2014; Hernandez-Viezcas et al., 2013). Plants cultivated hydroponically had NPs on their root surfaces (Martinez-Fernandez and Komarek, 2016). CeO2 NPs must physically come in contact with root secretions at the nanobeneficial interface for them to convert into ionic form in plants (Ma et al., 2015). The shape of NPs may also have an impact on conversion; NPs and nanocubes have higher surface reactivity than nanowires, leading to increased Ag+ release and toxicity (Gorka and Liu, 2016). Rod-shaped CeO2 NPs converted Ce3+ to a higher extent than other CeO2 NPs, demonstrating that rod-shaped NPs are the most chemically reactive (Zhang et al., 2017).
The transformation of NPs by plants has been extensively studied. Redox compounds are the main cause of NP transformation in plants. Accordingly, our examination of the biological concepts underpinning the impacts of NPs on plants will thus have a framework to work on. Additionally, this framework promotes the use and development of nanotechnology in agriculture, which could provide direction for further study.
The Effect of Nanomaterials on Metabolism in Plant
Often, more than one pathway participates in various aspects of plant metabolism. For example, glycolytic pathways and pentose phosphate pathways are involved in carbohydrate degradation; five different pathways participate in electron transfer in the respiratory chain, and electrons can be transferred to oxygen through a variety of terminal oxidases. Different metabolic pathways exist simultaneously in plants, but they do not operate at equal rates, and the ratio between rates is not fixed. Which metabolic pathway a plant employs in a given environment—and how different the pathways are—depends on the plant species, the organ, the stage of growth and development, and the environment.
The oxidative burst can be caused by NPs interfering with a variety of oxidative processes in plants, but they can also provide micronutrients, control gene expression, or perform all of these functions (Hossain et al., 2015; Liu and Lal, 2015; Nair and Chung, 2014). NPs can affect plant hormone levels. The increase in cytokinin level in pepper under Ag NPs stress and the decrease of IAA and ABA in cotton (Gossypium spp) under CuO NPs stress indicated that the NPs affect the hormone balance in plants, which in turn affect the metabolic processes in plants (O’Brien and Benkova, 2013). Following Y2O3 NPs stress, transcriptome analysis showed that genes involved in cell wall metabolism and remodeling undergo considerable alterations (Chen et al., 2021). NPs also influence ROS production, and electron transport in mitochondria and chloroplasts is affected by excess ROS. Photosynthesis is the only source of energy for plants; thus, mitochondria and chloroplasts have an impact on every aspect of plant metabolism and physiology (Foyer and Shigeoka, 2011; Kalaji et al., 2014). Based on the association between ROS and secondary signaling messengers, which results in transcriptional regulation of secondary metabolism, it has been determined that ROS produced during interactions with NPs may interfere with secondary metabolism in plants (Marslin et al., 2017). The metabolism of plants is similarly affected by the dosage effect of NPs. The secondary metabolism of the bean (Phaseolus vulgaris L.) roots and leaves was severely impacted at relatively low dosages (0, 25, 50, and 100 mg/L) of CeO2 NPs treatment (Salehi et al., 2020). In another report, carbon dots successfully improved soybean photosynthesis during a drought. The increase in photosynthesis had a big impact on agricultural yield and quality as well as growth and development (Wang et al., 2022a).
Generally speaking, NPs change the levels of hormones and ROS in plants, which affects their metabolic activities among the key physiological functions of plants. Studying the effects of NPs on plant metabolism can reveal the responses made by plants exposed to NPs.
In this review, we present the entry, migration, transformation, and metabolism of NPs in plants. The main factors affecting the migration transition of NPs are their size and surface charge. The transformation process of NPs is mainly caused by three factors, including (i) plant root secretions; (ii) biofilm interactions; and (iii) redox substances in plants. Current studies on size have found that nanoscale properties are predominant for particles below 30 nm. The current research on the size of NPs is getting more exhaustive. Plant hormone and ROS production may be impacted by NPs; plant hormones have an impact on a plant’s metabolism, and too many ROS prevent photosynthesis, which in turn affects metabolic processes in a plant.
Future research should consider the following points: (1) NMs may be modified to undergo surface modifications that alter the charge on their surface, facilitating the passage through organelle membranes; (2) Targeted transport of nanocarriers in plants is an interesting hypothesis (Miyamoto et al., 2022), which may provide good ideas for future targeted application of nanopesticides; (3) More studies need to examine the pathway and the amount of NPs entering the plant.
Author Contribution: Weichen Zhao designed the original idea and wrote the first draft; Pingfan Zhou, Benzhen Lou, Yaqi Jiang, Yuanbo Li, Mingshu Li, and Noman Shakoor contributed to the bibliographic search; Yukui Rui edited and reviewed the final version of the manuscript. All authors have approved the final version of the manuscript.
Ethics Approval: Not applicable.
Funding Statement: The project was supported by the National Key R&D Program of China (2017YFD0801300, 2017YFD0801103), the Key National Natural Science Foundation of China (No. 41130526), Professor workstation in Yuhuangmiao Town, Shanghe County, China Agricultural University, and Professor Workstation in Sunji Town, Shanghe County, China Agricultural University.
Conflicts of Interest: The authors declare that they have no conflicts of interest to report regarding the present study.
References
Adeel M, Farooq T, White JC, Hao Y, He ZF, Rui YK (2021). Carbon-based nanomaterials suppress tobacco mosaic virus (TMV) infection and induce resistance in Nicotiana benthamiana. Journal of Hazardous Materials 404: 10. DOI 10.1016/j.jhazmat.2020.124167. [Google Scholar] [CrossRef]
Avellan A, Schwab F, Masion A, Chaurand P, Borschneck D, Vidal V, Rose J, Santaella C, Levard C (2017). Nanoparticle uptake in plants: Gold nanomaterial localized in roots of arabidopsis thaliana by X-ray computed nanotomography and hyperspectral imaging. Environmental Science & Technology 51: 8682–8691. DOI 10.1021/acs.est.7b01133. [Google Scholar] [CrossRef]
Bai TH, Zhang P, Guo ZL, Chetwynd AJ, Zhang M et al. (2021). Different physiological responses of C3 and C4 plants to nanomaterials. Environmental Science and Pollution Research 28: 25542–25551. DOI 10.1007/s11356-021-12507-7. [Google Scholar] [CrossRef]
Bao WL, Wang JY, Wang Q, O’Hare D, Wan YL (2016). Layered double hydroxide nanotransporter for molecule delivery to intact plant cells. Scientific Reports 6: 26738. DOI 10.1038/srep26738. [Google Scholar] [CrossRef]
Bindraban PS, Dimkpa CO, Angle S, Rabbinge R (2018). Unlocking the multiple public good services from balanced fertilizers. Food Security 10: 273–285. DOI 10.1007/s12571-018-0769-4. [Google Scholar] [CrossRef]
Carpita N, Sabularse D, Montezinos D, Delmer DP (1979). Determination of the pore-size of cell-walls of living plant-cells. Science 205: 1144–1147. DOI 10.1126/science.205.4411.1144. [Google Scholar] [CrossRef]
Chen BF, Pan YZ, Chen YL, Zhang ZY, Yang ZH, Zheng M, Lu T, Jiang LY, Qian HF (2022). TiO2 nanoparticles exert an adverse effect on aquatic microbial communities. Science of the Total Environment 831: 9. DOI 10.1016/j.scitotenv.2022.154942. [Google Scholar] [CrossRef]
Chen FR, Wang CX, Yue L, Zhu LQ, Tang JF, Yu XY, Cao XS, Schroder P, Wang ZY (2021). Cell walls are remodeled to alleviate nY2O3 cytotoxicity by elaborate regulation of de novo synthesis and vesicular transport. ACS Nano 15: 13166–13177. DOI 10.1021/acsnano.1c02715. [Google Scholar] [CrossRef]
Chesson A, Gardner PT, Wood TJ (1997). Cell wall porosity and available surface area of wheat straw and wheat grain fractions. Journal of the Science of Food and Agriculture 75: 289–295. DOI 10.1002/(ISSN)1097-0010. [Google Scholar] [CrossRef]
Chong MN, Jin B, Chow CWK, Saint C (2010). Recent developments in photocatalytic water treatment technology: A review. Water Research 44: 2997–3027. DOI 10.1016/j.watres.2010.02.039. [Google Scholar] [CrossRef]
Chundawat SPS, Donohoe BS, Sousa LD, Elder T, Agarwal UP, Lu FC, Ralph J, Himmel ME, Balan V, Dale BE (2011). Multi-scale visualization and characterization of lignocellulosic plant cell wall deconstruction during thermochemical pretreatment. Energy & Environmental Science 4: 973–984. DOI 10.1039/c0ee00574f. [Google Scholar] [CrossRef]
Cui D, Zhang P, Ma YH, He X, Li YY, Zhang J, Zhao YC, Zhang ZY (2014). Effect of cerium oxide nanoparticles on asparagus lettuce cultured in an agar medium. Environmental Science-Nano 1: 459–465. DOI 10.1039/C4EN00025K. [Google Scholar] [CrossRef]
de la Rosa G, Lopez-Moreno ML, Hernandez-Viezcas J, Montes MO, Peralta-Videa JR, Gardea-Torresdey JL (2011). Toxicity and biotransformation of ZnO nanoparticles in the desert plants Prosopis juliflora-velutina, Salsola tragus and Parkinsonia florida. International Journal of Nanotechnology 8: 492–506. DOI 10.1504/IJNT.2011.040190. [Google Scholar] [CrossRef]
Dev A, Srivastava AK, Karmakar S (2018). Nanomaterial toxicity for plants. Environmental Chemistry Letters 16: 85–100. DOI 10.1007/s10311-017-0667-6. [Google Scholar] [CrossRef]
Dietz KJ, Herth S (2011). Plant nanotoxicology. Trends in Plant Science 16: 582–589. DOI 10.1016/j.tplants.2011.08.003. [Google Scholar] [CrossRef]
Dimkpa CO, McLean JE, Latta DE, Manangon E, Britt DW, Johnson WP, Boyanov MI, Anderson AJ (2012). CuO and ZnO nanoparticles: Phytotoxicity, metal speciation, and induction of oxidative stress in sand-grown wheat. Journal of Nanoparticle Research 14: 1125. DOI 10.1007/s11051-012-1125-9. [Google Scholar] [CrossRef]
Faizan M, Faraz A, Hayat S, Bhat JA, Yu FY (2021). Zinc oxide nanoparticles and epibrassinolide enhanced growth of tomato via modulating antioxidant activity and photosynthetic performance. BIOCELL 45: 1081–1093. DOI 10.32604/biocell.2021.015363. [Google Scholar] [CrossRef]
Fan RM, Huang YC, Grusak MA, Huang CP, Sherrier DJ (2014). Effects of nano-TiO2 on the agronomically-relevant Rhizobium-legume symbiosis. Science of the Total Environment 466: 503–512. DOI 10.1016/j.scitotenv.2013.07.032. [Google Scholar] [CrossRef]
Farooq T, Adeel M, He ZF, Umar M, Shakoor N, da Silva W, Elmer W, White JC, Rui YK (2021). Nanotechnology and plant viruses: An emerging disease management approach for resistant pathogens. ACS Nano 15: 6030–6037. DOI 10.1021/acsnano.0c10910. [Google Scholar] [CrossRef]
Fleischer A, O’Neill MA, Ehwald R (1999). The pore size of non-graminaceous plant cell walls is rapidly decreased by borate ester cross-linking of the pectic polysaccharide rhamnogalacturonan II. Plant Physiology 121: 829–838. DOI 10.1104/pp.121.3.829. [Google Scholar] [CrossRef]
Foyer CH, Shigeoka S (2011). Understanding oxidative stress and antioxidant functions to enhance photosynthesis. Plant Physiology 155: 93–100. DOI 10.1104/pp.110.166181. [Google Scholar] [CrossRef]
Fujino T, Itoh T (1998). Changes in pectin structure during epidermal cell elongation in pea (Pisum sativum) and its implications for cell wall architecture. Plant and Cell Physiology 39: 1315–1323. DOI 10.1093/oxfordjournals.pcp.a029336. [Google Scholar] [CrossRef]
Gao ST, Tang GS, Hua DW, Xiong RH, Han JQ, Jiang SH, Zhang QL, Huang CB (2019). Stimuli-responsive bio-based polymeric systems and their applications. Journal of Materials Chemistry B 7: 709–729. DOI 10.1039/C8TB02491J. [Google Scholar] [CrossRef]
Gardea-Torresdey JL, Gomez E, Peralta-Videa JR, Parsons JG, Troiani H, Jose-Yacaman M (2003). Alfalfa sprouts: A natural source for the synthesis of silver nanoparticles. Langmuir 19: 1357–1361. DOI 10.1021/la020835i. [Google Scholar] [CrossRef]
Gardea-Torresdey JL, Parsons JG, Gomez E, Peralta-Videa J, Troiani HE, Santiago P, Yacaman MJ (2002). Formation and growth of Au nanoparticles inside live alfalfa plants. Nano Letters 2: 397–401. DOI 10.1021/nl015673+. [Google Scholar] [CrossRef]
Gorka DE, Liu J (2016). Effect of direct contact on the phytotoxicity of silver nanomaterials. Environmental Science & Technology 50: 10370–10376. DOI 10.1021/acs.est.6b02434. [Google Scholar] [CrossRef]
Guo KR, Fu DH, Adeel M, Shen YZ, Wang KX, Hao Y, Bai TH, Wang YY, Rui YK (2022). Toxicities of nanomaterials and metals to rice under low atmospheric pressure. Acta Physiologiae Plantarum 44: 58. DOI 10.1007/s11738-022-03391-w. [Google Scholar] [CrossRef]
Hernandez-Viezcas JA, Castillo-Michel H, Andrews JC, Cotte M, Rico C, Peralta-Videa JR, Ge Y, Priester JH, Holden PA, Gardea-Torresdey JL (2013). In situ synchrotron X-ray fluorescence mapping and speciation of CeO2 and ZnO nanoparticles in soil cultivated soybean (glycine max). ACS Nano 7: 1415–1423. DOI 10.1021/nn305196q. [Google Scholar] [CrossRef]
Hernandez-Viezcas JA, Castillo-Michel H, Servin AD, Peralta-Videa JR, Gardea-Torresdey JL (2011). Spectroscopic verification of zinc absorption and distribution in the desert plant Prosopis juliflora-velutina (velvet mesquite) treated with ZnO nanoparticles. Chemical Engineering Journal 170: 346–352. DOI 10.1016/j.cej.2010.12.021. [Google Scholar] [CrossRef]
Hossain Z, Mustafa G, Komatsu S (2015). Plant responses to nanoparticle stress. International Journal of Molecular Sciences 16: 26644–26653. DOI 10.3390/ijms161125980. [Google Scholar] [CrossRef]
Houston K, Tucker MR, Chowdhury J, Shirley N, Little A (2016). The plant cell wall: A complex and dynamic structure as revealed by the responses of genes under stress conditions. Frontiers in Plant Science 7: 784. DOI 10.3389/fpls.2016.00984. [Google Scholar] [CrossRef]
Hu P, An J, Faulkner MM, Wu H, Li Z, Tian X, Giraldo JP (2020). Nanoparticle charge and size control foliar delivery efficiency to plant cells and organelles. ACS Nano 14: 7970–7986. DOI 10.1021/acsnano.9b09178. [Google Scholar] [CrossRef]
Hua DW, Harizaj A, Wels M, Brans T, Stremersch S et al. (2021). Bubble forming films for spatial selective cell killing. Advanced Materials 33: 2008379. DOI 10.1002/Adma.202008379. [Google Scholar] [CrossRef]
Iannone MF, Groppa MD, Benavides MP (2014). Effect of magnetite iron oxide nanoparticles on wheat plants. BIOCELL 38: 169. [Google Scholar]
Jia H, Ma P, Huang L, Wang X, Chen C, Liu C, Wei T, Yang J, Guo J, Li J (2022). Hydrogen sulphide regulates the growth of tomato root cells by affecting cell wall biosynthesis under CuO NPs stress. Plant Biology 24: 627–635. DOI 10.1111/plb.13316. [Google Scholar] [CrossRef]
Jiang Y, Zhou P, Zhang P, Adeel M, Shakoor N et al. (2022). Green synthesis of metal-based nanoparticles for sustainable agriculture. Environmental Pollution 309: 119755. DOI 10.1016/j.envpol.2022.119755. [Google Scholar] [CrossRef]
Jjagwe J, Olupot PW, Menya E, Kalibbala HM (2021). Synthesis and application of granular activated carbon from biomass waste materials for water treatment: A review. Journal of Bioresources and Bioproducts 6: 292–322. DOI 10.1016/j.jobab.2021.03.003. [Google Scholar] [CrossRef]
Juarez-Maldonado A (2022). Impact of nanomaterials on plants: What other implications do they have? BIOCELL 46: 651–654. DOI 10.32604/biocell.2022.017350. [Google Scholar] [CrossRef]
Juarez-Maldonado A, Ortega-Ortiz H, Morales-Diaz AB, Gonzalez-Morales S, Morelos-Moreno A, Cabrera-De la Fuente M, Sandoval-Rangel A, Cadenas-Pliego G, Benavides-Mendoza A (2019). Nanoparticles and nanomaterials as plant biostimulants. International Journal of Molecular Sciences 20: 162. DOI 10.3390/ijms20010162. [Google Scholar] [CrossRef]
Juárez-Maldonado A (2022). Impact of nanomaterials on plants: What other implications do they have? BIOCELL 46: 651–654. DOI 10.32604/biocell.2022.017350. [Google Scholar] [CrossRef]
Kalaji HM, Schansker G, Ladle RJ, Goltsev V, Bosa K et al. (2014). Frequently asked questions about in vivo chlorophyll fluorescence: Practical issues. Photosynthesis Research 122: 121–158. DOI 10.1007/s11120-014-0024-6. [Google Scholar] [CrossRef]
Khan MF, Ansari AH, Hameedullah M, Ahmad E, Husain FM et al. (2016). Sol-gel synthesis of thorn-like ZnO nanoparticles endorsing mechanical stirring effect and their antimicrobial activities: Potential role as nano-antibiotics. Scientific Reports 6: 27689. DOI 10.1038/srep27689. [Google Scholar] [CrossRef]
Kim JH, Lee Y, Kim EJ, Gu S, Sohn EJ, Seo YS, An HJ, Chang YS (2014). Exposure of iron nanoparticles to Arabidopsis thaliana enhances root elongation by triggering cell wall loosening. Environmental Science & Technology 48: 3477–3485. DOI 10.1021/es4043462. [Google Scholar] [CrossRef]
Larue C, Castillo-Michel H, Sobanska S, Cecillon L, Bureau S, Barthes V, Ouerdane L, Carriere M, Sarret G (2014). Foliar exposure of the crop Lactuca sativa to silver nanoparticles: Evidence for internalization and changes in Ag speciation. Journal of Hazardous Materials 264: 98–106. DOI 10.1016/j.jhazmat.2013.10.053. [Google Scholar] [CrossRef]
Lew TTS, Wong MH, Kwak SY, Sinclair R, Koman VB, Strano MS (2018). Rational design principles for the transport and subcellular distribution of nanomaterials into plant protoplasts. Small 14: 1802086. DOI 10.1002/smll.201802086. [Google Scholar] [CrossRef]
Li J, Hu J, Ma C, Wang Y, Wu C, Huang J, Xing B (2016). Uptake, translocation and physiological effects of magnetic iron oxide (γ-Fe2O3) nanoparticles in corn (Zea mays L.). Chemosphere 159: 326–334. DOI 10.1016/j.chemosphere.2016.05.083. [Google Scholar] [CrossRef]
Li MS, Zhang P, Adeel M, Guo ZL, Chetwynd AJ, Ma CX, Bai TH, Hao Y, Rui YK (2021). Physiological impacts of zero valent iron, Fe3O4 and Fe2O3 nanoparticles in rice plants and their potential as Fe fertilizers. Environmental Pollution 269: 116134. DOI 10.1016/j.envpol.2020.116134. [Google Scholar] [CrossRef]
Li M, Zhang YZ, Feng S, Zhang XX, Xi YL, Xiang XL (2022). Bioaccumulation and biomagnification effects of nano-TiO2 in the aquatic food chain. Ecotoxicology 31: 1023–1034. DOI 10.1007/s10646-022-02572-0. [Google Scholar] [CrossRef]
Lin SJ, Reppert J, Hu Q, Hudson JS, Reid ML, Ratnikova TA, Rao AM, Luo H, Ke PC (2009). Uptake, translocation, and transmission of carbon nanomaterials in rice plants. Small 5: 1128–1132. DOI 10.1002/smll.200801556. [Google Scholar] [CrossRef]
Line C, Reyes-Herrera J, Bakshi M, Wazne M, Costa V, Roujol D, Jamet E, Castillo-Michel H, Flahaut E, Larue C (2021). Fourier transform infrared spectroscopy contribution to disentangle nanomaterial (DWCNT, TiO2) impacts on tomato plants. Environmental Science-Nano 8: 2920–2931. DOI 10.1039/d1en00455g. [Google Scholar] [CrossRef]
Liu RQ, Lal R (2015). Potentials of engineered nanoparticles as fertilizers for increasing agronomic productions. Science of the Total Environment 514: 131–139. DOI 10.1016/j.scitotenv.2015.01.104. [Google Scholar] [CrossRef]
Liu JH, Li GJ, Chen LL, Gu JJ, Wu HH, Li ZH (2021). Cerium oxide nanoparticles improve cotton salt tolerance by enabling better ability to maintain cytosolic K+/Na+ ratio. Journal of Nanobiotechnology 19: 153. DOI 10.1186/s12951-021-00892-7. [Google Scholar] [CrossRef]
Lopez-Moreno ML, de la Rosa G, Hernandez-Viezcas JA, Castillo-Michel H, Botez CE, Peralta-Videa JR, Gardea-Torresdey JL (2010). Evidence of the differential biotransformation and genotoxicity of ZnO and CeO2 nanoparticles on soybean (glycine max) Plants. Environmental Science & Technology 44: 7315–7320. DOI 10.1021/es903891g. [Google Scholar] [CrossRef]
Lou BZ, Shakoor N, Adeel M, Zhang P, Huang LL, Zhao YW, Zhao WC, Jiang YQ, Rui YK (2022). Catalytic oxidation of volatile organic compounds by non-noble metal catalyst: Current advancement and future prospectives. Journal of Cleaner Production 363: 132523. DOI 10.1016/j.jclepro.2022.132523. [Google Scholar] [CrossRef]
Lv JT, Christie P, Zhang SZ (2019). Uptake, translocation, and transformation of metal-based nanoparticles in plants: Recent advances and methodological challenges. Environmental Science-Nano 6: 41–59. DOI 10.1039/C8EN00645H. [Google Scholar] [CrossRef]
Lv ZY, Sun HD, Du W, Li RY, Mao H, Kopittke PM (2021). Interaction of different-sized ZnO nanoparticles with maize (Zea mays) accumulation, biotransformation and phytotoxicity. Science of the Total Environment 796: 148927. DOI 10.1016/j.scitotenv.2021.148927. [Google Scholar] [CrossRef]
Lv JT, Zhang SZ, Luo L, Zhang J, Yang K, Christie P (2015). Accumulation, speciation and uptake pathway of ZnO nanoparticles in maize. Environmental Science-Nano 2: 68–77. DOI 10.1039/C4EN00064A. [Google Scholar] [CrossRef]
Ma YH, Zhang P, Zhang ZY, He X, Zhang JZ et al. (2015). Where does the transformation of precipitated ceria nanoparticles in hydroponic plants take place? Environmental Science & Technology 49: 10667–10674. DOI 10.1021/acs.est.5b02761. [Google Scholar] [CrossRef]
Marslin G, Sheeba CJ, Franklin G (2017). Nanoparticles alter secondary metabolism in plants via ROS burst. Frontiers in Plant Science 8: 832. DOI 10.3389/fpls.2017.00832. [Google Scholar] [CrossRef]
Martinez-Fernandez D, Komarek M (2016). Comparative effects of nanoscale zero-valent iron (nZVI) and Fe2O3 nanoparticles on root hydraulic conductivity of Solanum lycopersicum L. Environmental and Experimental Botany 131: 128–136. DOI 10.1016/j.envexpbot.2016.07.010. [Google Scholar] [CrossRef]
Milewska-Hendel A, Zubko M, Stroz D, Kurczynska EU (2019). Effect of nanoparticles surface charge on the Arabidopsis thaliana (L.) roots development and their movement into the root cells and protoplasts. International Journal of Molecular Sciences 20: 1650. DOI 10.3390/ijms20071650. [Google Scholar] [CrossRef]
Miyamoto T, Tsuchiya K, Toyooka K, Goto Y, Tateishi A, Numata K (2022). Relaxation of the plant cell wall barrier via zwitterionic liquid pretreatment for micelle-complex-mediated DNA delivery to specific plant organelles. Angewandte Chemie-International Edition 61: e202204234. DOI 10.1002/anie.202204234. [Google Scholar] [CrossRef]
Molnár Á., Rónavári A, Bélteky P, Szőllősi R, Valyon E, Oláh D, Rázga Z, Ördög A, Kónya Z, Kolbert Z (2020). ZnO nanoparticles induce cell wall remodeling and modify ROS/RNS signalling in roots of Brassica seedlings. Ecotoxicology and Environmental Safety 206: 111158. DOI 10.1016/j.ecoenv.2020.111158. [Google Scholar] [CrossRef]
Moore MN (2006). Do nanoparticles present ecotoxicological risks for the health of the aquatic environment? Environment International 32: 967–976. DOI 10.1016/j.envint.2006.06.014. [Google Scholar] [CrossRef]
Nair PMG, Chung IM (2014). Impact of copper oxide nanoparticles exposure on Arabidopsis thaliana growth, root system development, root lignificaion, and molecular level changes. Environmental Science and Pollution Research 21: 12709–12722. DOI 10.1007/s11356-014-3210-3. [Google Scholar] [CrossRef]
O’Brien JA, Benkova E (2013). Cytokinin cross-talking during biotic and abiotic stress responses. Frontiers in Plant Science 4: 451. DOI 10.3389/fpls.2013.00451. [Google Scholar] [CrossRef]
Pang LJ, Adeel M, Shakoor N, Guo KR, Ma DF, Ahmad MA, Lu GQ, Zhao MH, Li SE, Rui YK (2021). Engineered nanomaterials suppress the soft rot disease (Rhizopus stolonifer) and slow down the loss of nutrient in sweet potato. Nanomaterials 11: 2572. DOI 10.3390/nano11102572. [Google Scholar] [CrossRef]
Peng C, Duan DC, Xu C, Chen YS, Sun LJ et al. (2015). Translocation and biotransformation of CuO nanoparticles in rice (Oryza sativa L.) plants. Environmental Pollution 197: 99–107. DOI 10.1016/j.envpol.2014.12.008. [Google Scholar] [CrossRef]
Perozo E, Cortes DM, Sompornpisut P, Kloda A, Martinac B (2002). Open channel structure of MscL and the gating mechanism of mechanosensitive channels. Nature 418: 942–948. DOI 10.1038/nature00992. [Google Scholar] [CrossRef]
Pinheiro SKD, Miguel T, Chaves MD, Barros FCD, Farias CP, de Moura TA, Ferreira OP, Paschoal AR, Souza AG, Miguel ED (2021). Silver nanoparticles (AgNPs) internalization and passage through the Lactuca sativa (Asteraceae) outer cell wall. Functional Plant Biology 48: 1113–1123. DOI 10.1071/FP21161. [Google Scholar] [CrossRef]
Qu QL, Zhang XL, Ravanbakhsh H, Tang GS, Zhang J, Deng YK, Braeckmans K, De Smedt SC, Xiong RH, Huang CB (2022). Gas-shearing synthesis of core-shell multicompartmental microparticles as cell-like system for enzymatic cascade reaction. Chemical Engineering Journal 428: 132607. DOI 10.1016/j.cej.2021.132607. [Google Scholar] [CrossRef]
Salehi H, Miras-Moreno B, Rad AC, Pii Y, Mimmo T, Cesco S, Lucini L (2020). Relatively low dosages of CeO2 nanoparticles in the solid medium induce adjustments in the secondary metabolism and lonomic balance of bean (Phaseolus vulgaris L.) roots and leaves. Journal of Agricultural and Food Chemistry 68: 67–76. DOI 10.1021/acs.jafc.9b05107. [Google Scholar] [CrossRef]
Sardoiwala MN, Kaundal B, Choudhury SR (2018). Toxic impact of nanomaterials on microbes, plants and animals. Environmental Chemistry Letters 16: 147–160. DOI 10.1007/s10311-017-0672-9. [Google Scholar] [CrossRef]
Schwab F, Zhai GS, Kern M, Turner A, Schnoor JL, Wiesner MR (2016). Barriers, pathways and processes for uptake, translocation and accumulation of nanomaterials in plants–critical review. Nanotoxicology 10: 257–278. DOI 10.3109/17435390.2015.1048326. [Google Scholar] [CrossRef]
Schwabe F, Tanner S, Schulin R, Rotzetter A, Stark W, von Quadt A, Nowack B (2015). Dissolved cerium contributes to uptake of Ce in the presence of differently sized CeO2-nanoparticles by three crop plants. Metallomics 7: 466–477. DOI 10.1039/C4MT00343H. [Google Scholar] [CrossRef]
Schymura S, Fricke T, Hildebrand H, Franke K (2017). Elucidating the role of dissolution in CeO2 nanoparticle plant uptake by smart radiolabeling. Angewandte Chemie-International Edition 56: 7411–7414. DOI 10.1002/anie.201702421. [Google Scholar] [CrossRef]
Shakoor N, Adeel M, Zain M, Zhang P, Ahmad MA et al. (2022). Exposure of cherry radish (Raphanus sativus L. var. Radculus Pers) to iron-based nanoparticles enhances its nutritional quality by trigging the essential elements. Nanoimpact 25: 100388. DOI 10.1016/j.impact.2022.100388. [Google Scholar] [CrossRef]
Sharifi S, Behzadi S, Laurent S, Forrest ML, Stroeve P, Mahmoudi M (2012). Toxicity of nanomaterials. Chemical Society Reviews 41: 2323–2343. DOI 10.1039/C1CS15188F. [Google Scholar] [CrossRef]
Shomer I, Novacky AJ, Pike SM, Yermiyahu U, Kinraide TB (2003). Electrical potentials of plant cell walls in response to the ionic environment. Plant Physiology 133: 411–422. DOI 10.1104/pp.103.024539. [Google Scholar] [CrossRef]
Siddiqi KS, Husen A (2017). Plant response to engineered metal oxide nanoparticles. Nanoscale Research Letters 12: 92. DOI 10.1186/s11671-017-1861-y. [Google Scholar] [CrossRef]
Singh A, Singh NB, Afzal S, Singh T, Hussain I (2018). Zinc oxide nanoparticles: a review of their biological synthesis, antimicrobial activity, uptake, translocation and biotransformation in plants. Journal of Materials Science 53: 185–201. DOI 10.1007/s10853-017-1544-1. [Google Scholar] [CrossRef]
Sosan A, Svistunenko D, Straltsova D, Tsiurkina K, Smolich I et al. (2016). Engineered silver nanoparticles are sensed at the plasma membrane and dramatically modify the physiology of Arabidopsis thaliana plants. Plant Journal 85: 245–257. DOI 10.1111/tpj.13105. [Google Scholar] [CrossRef]
Usman M, Farooq M, Wakeel A, Nawaz A, Cheema SA, Rehman HU, Ashraf I, Sanaullah M (2020). Nanotechnology in agriculture: Current status, challenges and future opportunities. Science of the Total Environment 721: 16. DOI 10.1016/j.scitotenv.2020.137778. [Google Scholar] [CrossRef]
Verma SK, Das AK, Gantait S, Kumar V, Gurel E (2019). Applications of carbon nanomaterials in the plant system: A perspective view on the pros and cons. Science of the Total Environment 667: 485–499. DOI 10.1016/j.scitotenv.2019.02.409. [Google Scholar] [CrossRef]
Wagner G, Korenkov V, Judy JD, Bertsch PM (2016). Nanoparticles composed of Zn and ZnO inhibit Peronospora tabacina spore germination in vitro and P. tabacina infectivity on tobacco leaves. Nanomaterials 6: 50. DOI 10.3390/nano6030050. [Google Scholar] [CrossRef]
Wang Y, Deng C, Elmer WH, Dimkpa CO, Sharma S et al. (2022d). Therapeutic delivery of nanoscale sulfur to suppress disease in tomatoes: In Vitro imaging and orthogonal mechanistic investigation. ACS Nano 16: 11204–11217. DOI 10.1021/acsnano.2c04073. [Google Scholar] [CrossRef]
Wang C, Ji Y, Cao X, Yue L, Chen F, Li J, Yang H, Wang Z, Xing B (2022a). Carbon dots improve nitrogen bioavailability to promote the growth and nutritional quality of soybeans under drought stress. ACS Nano 16: 12415-12424. DOI 10.1021/acsnano.2c03591. [Google Scholar] [CrossRef]
Wang YY, Jiang FP, Ma CX, Rui YK, Tsang DCW, Xing BS (2019). Effect of metal oxide nanoparticles on amino acids in wheat grains (Triticum aestivum) in a life cycle study. Journal of Environmental Management 241: 319–327. DOI 10.1016/j.jenvman.2019.04.041. [Google Scholar] [CrossRef]
Wang CX, Liu XF, Chen FR, Yue L, Cao XS, Li J, Cheng BX, Wang ZY, Xing BS (2022b). Selenium content and nutritional quality of Brassica chinensis L enhanced by selenium engineered nanomaterials: The role of surface charge. Environmental Pollution 308: 119582. DOI 10.1016/j.envpol.2022.119582. [Google Scholar] [CrossRef]
Wang P, Menzies NW, Lombi E, McKenna BA, Johannessen B, Glover CJ, Kappen P, Kopittke PM (2013). Fate of ZnO nanoparticles in soils and cowpea (Vigna unguiculata). Environmental Science & Technology 47: 13822–13830. DOI 10.1021/es403466p. [Google Scholar] [CrossRef]
Wang DJ, Saleh NB, Byro A, Zepp R, Sahle-Demessie E et al. (2022c). Nano-enabled pesticides for sustainable agriculture and global food security. Nature Nanotechnology 17: 347–360. DOI 10.1038/s41565-022-01082-8. [Google Scholar] [CrossRef]
Wang ZY, Xie XY, Zhao J, Liu XY, Feng WQ, White JC, Xing BS (2012). Xylem- and phloem-based transport of CuO nanoparticles in maize (Zea mays L.). Environmental Science & Technology 46: 4434–4441. DOI 10.1021/es204212z. [Google Scholar] [CrossRef]
Wang YY, Zhang P, Li MS, Guo ZL, Ullah S, Rui YK, Lynch I (2020). Alleviation of nitrogen stress in rice (Oryza sativa) by ceria nanoparticles. Environmental Science-Nano 7: 2930–2940. DOI 10.1039/D0EN00757A. [Google Scholar] [CrossRef]
Wong MH, Misra RP, Giraldo JP, Kwak SY, Son Y, Landry MP, Swan JW, Blankschtein D, Strano MS (2016). Lipid exchange envelope penetration (LEEP) of nanoparticles for plant engineering: A universal localization mechanism. Nano Letters 16: 1161–1172. DOI 10.1021/acs.nanolett.5b04467. [Google Scholar] [CrossRef]
Wu H, Li Z (2022). Nano-enabled agriculture: how nanoparticles cross barriers in plants? Plant Communications 6: 100346. DOI 10.1016/j.xplc.2022.100346. [Google Scholar] [CrossRef]
Wu HH, Shabala L, Barry K, Zhou MX, Shabala S (2013). Ability of leaf mesophyll to retain potassium correlates with salinity tolerance in wheat and barley. Physiologia Plantarum 149: 515–527. DOI 10.1111/ppl.12056. [Google Scholar] [CrossRef]
Wu HH, Tito N, Giraldo JP (2017). Anionic cerium oxide nanoparticles protect plant photosynthesis from abiotic stress by scavenging reactive oxygen species. ACS Nano 11: 11283–11297. DOI 10.1021/acsnano.7b05723. [Google Scholar] [CrossRef]
Yin LY, Cheng YW, Espinasse B, Colman BP, Auffan M, Wiesner M, Rose J, Liu J, Bernhardt ES (2011). More than the ions: The effects of silver nanoparticles on Lolium multiflorum. Environmental Science & Technology 45: 2360–2367. DOI 10.1021/es103995x. [Google Scholar] [CrossRef]
Yue L, Feng Y, Ma CX, Wang CX, Chen FR, Cao XS, Wang J, White JC, Wang ZY, Xing BS (2022). Molecular mechanisms of early flowering in tomatoes induced by manganese ferrite (MnFe2O4) nanomaterials. ACS Nano 16: 5636–5646. DOI 10.1021/acsnano.1c10602. [Google Scholar] [CrossRef]
Yusefi-Tanha E, Fallah S, Rostamnejadi A, Pokhrel LR (2022). Responses of soybean (Glycine max L. Merr.) to zinc oxide nanoparticles: Understanding changes in root system architecture, zinc tissue partitioning and soil characteristics. Science of the Total Environment 835: 155348. DOI 10.1016/j.scitotenv.2022.155348. [Google Scholar] [CrossRef]
Zeng Y, Himmel ME, Ding SY (2017). Visualizing chemical functionality in plant cell walls. Biotechnology for Biofuels 10: 263. DOI 10.1186/s13068-017-0953-3. [Google Scholar] [CrossRef]
Zhang J, Guo WL, Li QQ, Wang Z, Liu SJ (2018). The effects and the potential mechanism of environmental transformation of metal nanoparticles on their toxicity in organisms. Environmental Science-Nano 5: 19. DOI 10.1039/c8en00688a. [Google Scholar] [CrossRef]
Zhang P, Ma YH, Zhang ZY, He X, Li YY, Zhang J, Zheng LR, Zhao YL (2015). Species-specific toxicity of ceria nanoparticles to Lactuca plants. Nanotoxicology 9: 1–8. DOI 10.3109/17435390.2013.855829. [Google Scholar] [CrossRef]
Zhang P, Ma YH, Zhang ZY, He X, Zhang J, Guo Z, Tai RZ, Zhao YL, Chai ZF (2012). Biotransformation of ceria nanoparticles in cucumber plants. ACS Nano 6: 9943–9950. DOI 10.1021/nn303543n. [Google Scholar] [CrossRef]
Zhang P, Xie CJ, Ma YH, He X, Zhang ZY, Ding YY, Zheng LR, Zhang J (2017). Shape-dependent transformation and translocation of ceria nanoparticles in cucumber plants. Environmental Science & Technology Letters 4: 380–385. DOI 10.1021/acs.estlett.7b00359. [Google Scholar] [CrossRef]
Zhao WC, Adeel M, Zhang P, Zhou PF, Huang LL et al. (2022b). A critical review on surface-modified nano-catalyst application for the photocatalytic degradation of volatile organic compounds. Environmental Science-Nano 9: 61–80. DOI 10.1039/D1EN00955A. [Google Scholar] [CrossRef]
Zhao W, Liu Y, Zhang P, Zhou P, Wu Z et al. (2022a). Engineered Zn-based nano-pesticides as an opportunity for treatment of phytopathogens in agriculture. NanoImpact 28: 100420. DOI 10.1016/j.impact.2022.100420. [Google Scholar] [CrossRef]
Zhao LJ, Lu L, Wang AD, Zhang HL, Huang M, Wu HH, Xing BS, Wang ZY, Ji R (2020). Nano-biotechnology in agriculture: Use of nanomaterials to promote plant growth and stress tolerance. Journal of Agricultural and Food Chemistry 68: 1935–1947. DOI 10.1021/acs.jafc.9b06615. [Google Scholar] [CrossRef]
Zhou P, Adeel M, Shakoor N, Guo M, Hao Y, Azeem I, Li M, Liu M, Rui Y (2021b). Application of nanoparticles alleviates heavy metals stress and promotes plant growth: An overview. Nanomaterials 11: 26. DOI 10.3390/nano11010026. [Google Scholar] [CrossRef]
Zhou H, Wu HH, Zhang F, Su Y, Guan WX, Xie YJ, Giraldo JP, Shen WB (2021a). Molecular basis of cerium oxide nanoparticle enhancement of rice salt tolerance and yield. Environmental Science-Nano 8: 3294–3311. DOI 10.1039/D1EN00390A. [Google Scholar] [CrossRef]
Zhu JH, Wang J, Zhan XH, Li AZ, White JC, Gardea-Torresdey JL, Xing BS (2021). Role of charge and size in the translocation and distribution of zinc oxide particles in wheat cells. ACS Sustainable Chemistry & Engineering 9: 11556–11564. DOI 10.1021/acssuschemeng.1c04080. [Google Scholar] [CrossRef]
Cite This Article
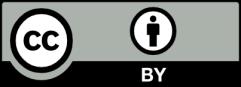
This work is licensed under a Creative Commons Attribution 4.0 International License , which permits unrestricted use, distribution, and reproduction in any medium, provided the original work is properly cited.