Open Access
ARTICLE
Maternal hyperthyroidism increases the synthesis activity and the osteogenic markers expression of calvarial osteoblasts from offspring in a murine model
1 Núcleo de Células Tronco e Terapia Celular Animal (NCT-TCA), Veterinay School, Universidade Federal de Minas Gerais, Belo Horizonte, Minas Gerais, 31270-901, Brasil
2 Universidade de Uberaba (UNIUBE), Uberaba, Minas Gerais, 38065-300, Brasil
3 Instituto de Ciências Biológicas, Universidade Federal de Minas Gerais, Belo Horizonte, Minas Gerais, 31270-901, Brasil
4 Universidade Estadual de Santa Cruz, Campus Soane Nazare de Andrade, Ilheus, Bahia, Brasil
* Corresponding Author: ROGéRIA SERAKIDES. Email:
BIOCELL 2023, 47(2), 423-430. https://doi.org/10.32604/biocell.2023.023689
Received 09 May 2022; Accepted 08 August 2022; Issue published 18 November 2022
Abstract
To evaluate the characteristics and synthesis activity of osteoblasts extracted from the calvaria of offspring of rats exposed to maternal hyperthyroidism. Twelve adult Wistar rats were divided into two groups, one control and one treated with daily administration of L-thyroxine by an orogastric tube (50 µg/animal/day) during pregnancy. Three days after delivery and confirmation of the mothers’ hyperthyroidism, the offspring were euthanized for the extraction of osteoblasts from the calvaria. At 7, 14, and 21 days, proliferation activity was assessed using MTT assay, while alkaline phosphatase (ALP) activity was assessed by the BCIP/NBT method. At 21 days, the total area of the mineralized matrix stained by von Kossa was evaluated by morphometry. The expression of gene transcripts for Runx2, Bmp2, Fgfr1, collagen type 1 (Col1), osteocalcin (Oc), and osteopontin (Op) were evaluated by real-time RT-PCR. Means were compared using the Student’s t-test. FA activity was significantly higher at 14 and 21 days in cultures of osteoblasts extracted from offspring exposed to maternal hyperthyroidism, while MTT conversion was significantly lower at 21 days in this group. Osteoblast cultures of neonates exposed to maternal hyperthyroidism also showed a larger total area of mineralized matrix and greater expression of gene transcripts for Oc and Op. Maternal hyperthyroidism increases the activity of matrix synthesis, alkaline phosphatase activity, and expression of gene transcripts for osteocalcin and osteopontin in the osteoblasts, extracted from the calvaria of the offspring, which may be one of the mechanisms of premature fusion of cranial sutures.Keywords
Thyroid dysfunction can occur at any stage of life, but is common during pregnancy. Maternal hyperthyroidism has an estimated incidence of 0.2%, reaching up to 2.5% when cases of subclinical maternal hyperthyroidism are considered (Taylor et al., 2018). The two main causes of hyperthyroidism during pregnancy are Graves’ disease and transient gestational thyrotoxicosis, resulting from elevated human chorionic gonadotropin (HCG)(Krassas et al., 2010).
Under normal conditions, thyroid hormones (THs), represented by triiodothyronine (T3) and thyroxine (T4), cross the placenta into the fetal circulation in sufficient quantity to support fetal thyroid function, having critical action in all systems, particularly in bone formation and growth (Brent, 2000; Neale et al., 2007; Gouveia et al., 2018). However, excess thyroxine from the mother during pregnancy and lactation (maternal hyperthyroidism) causes several changes in the bones of animals and children (Springer et al., 2017; Andersen and Andersen, 2021).
Excess maternal thyroxine reduces the longitudinal growth of long bones, such as the femur and humerus, in rat pups at birth and weaning (Maia et al., 2016). Reduced endochondral bone growth in rats caused by maternal hyperthyroidism is associated with increased chondrocyte hypertrophy and reduced expression of angiogenic factors, such as vascular endothelial growth factor (Vegf), vascular endothelial receptor-2 (Flk-1), and tyrosine kinase receptor 2 (Tie2) (Ribeiro et al., 2018a, 2018b). The pathogenesis of endochondral growth alterations resulting from hyperthyroidism has been widely studied (Maia et al., 2016; Ribeiro et al., 2018a, 2018b) when compared to intramembranous growth alterations (Howie et al., 2016).
Intramembranous ossification is responsible for the formation of the skullcap or calvaria, scapula, ilium, and part of the clavicle (Leitch et al., 2020); however, the main consequences of excess thyroid hormones are observed in the skull (Carmichael et al., 2015), which is therefore the main study site. Craniofacial development begins at approximately four weeks of gestation in humans, with the distinct condensations of the mesenchyme (Leitch et al., 2020). Condensation and formation of intramembranous ossification centers occur continuously, forming bones that are called bony spikes, which in turn radiate progressively from the primary ossification centers towards the periphery, leading to the formation of immature trabecular bone, which is gradually replaced by the cortical bone, during birth (Jin et al., 2016). After birth, the cranial bones do not fuse immediately, remaining separated by sutures and fontanelles that allow the skull to expand during growth (Opperman, 2000; Leitch et al., 2020). The molecular mechanisms that regulate craniofacial development and postnatal growth depend on several signaling pathways that have not yet been fully elucidated. However, some of the main transcription factors involved have already been identified (Marie, 2008; Leitch et al., 2020).
Runx2 is essential for the differentiation of mesenchymal stem cells (MSCs) into osteoblasts, since inactivation of Runx2 inhibits the differentiation of MSCs into osteoblasts, with complete absence of intramembranous differentiation (Komori, 2020). In addition, Runx2 regulates several genes in osteoblasts, such as bone sialoprotein (SPO), osteopontin (OP), osteocalcin (OC), and type I collagen (COL1), that play an important role in the formation of the bone matrix (Marie, 2008). The expression and activity of transcription factors responsible for bone formation are regulated by hormones and growth factors, such as bone morphogenetic protein (BMP), fibroblast growth factor (FGF), and transforming growth factor beta (TGFβ) (Marie, 2008).
Although the effect of thyroid hormones on intramembranous ossification is poorly understood, there are several reports on bone changes, mainly craniosynostosis due to maternal hyperthyroidism (de Lima et al., 1999; Rasmussen et al., 2007) or hyperthyroidism acquired during growth in animals (O’Shea et al., 2003, 2005; Bassett et al., 2007) and children (Johnsonbaugh et al., 1978; Segni et al., 1999). Craniosynostosis is the premature fusion of one or more cranial sutures before the cessation of brain growth, which can lead to facial deformities and brain damage due to increased intracranial pressure (Twigg and Wilkie, 2015). Puppies of mice exposed to maternal hyperthyroidism have altered craniofacial morphology, with fusion of premature coronal suture and widening by compensatory growth of the sagittal suture, with high expression of Runx2 and alkaline phosphatase (Howie et al., 2016). In vitro, the addition of thyroxine to cultures of osteoblasts extracted from the calvaria of mice increased the expression of osteogenic markers such as alkaline phosphatase and osteocalcin at seven days of culture (Cray et al., 2013). In vitro studies with the addition of thyroxine in the culture medium do not consider the transformation of T4 into T3, the placental passage of the hormone, among other metabolic pathways. Therefore, the present study extracts osteoblasts from neonates born to mothers with hyperthyroidism.
To the best of our knowledge, this is the first study to investigate the synthesis activity and phenotype of osteoblasts extracted from the calvaria of rats exposed to maternal hyperthyroidism. The aim was to verify whether osteoblasts extracted from the calvaria of newborn rats exposed to maternal hyperthyroidism could present greater activity and be responsible for the premature fusion of the sutures. We hypothesized that craniosynostosis resulting from maternal hyperthyroidism occurs due to an increase in the synthesis activity and in the osteogenic markers expression of calvarial osteoblasts. Osteoblasts were subjected to measurement of MTT conversion, alkaline phosphatase, total area of mineralized matrix, and expression of gene transcripts for Runx2, collagen type I, Bmp2, Fgfr1, osteocalcin, and osteopontin, by real-time quantitative RT-PCR (RT-qPCR).
All procedures were approved by the Ethics Committee on Animal Use of the Federal University of Minas Gerais (protocol number 156/2020).
Mating and administration of thyroxine
Twelve two-month-old adult female Wistar rats were obtained from Central Vivarium at University Federal of Minas Gerais. All animals used in this study were specific pathogen-free (SPF).
Animals are housed at a density of four rats per cage on a 12-h light-dark cycle and received food and water ad libitum. The females of all groups were submitted to vaginal cytology and the rats in proestrus were housed in plastic cages with adult male rats for 12 h during the night. The next morning, vaginal smears were performed and gestation was confirmed by the presence of sperm in the vaginal cytology. That day was designated as gestation day 0, and rats were separated, randomly, into individual boxes, comprising the treated (n = 6) and control groups (n = 6).
The treated rats received daily administration of L-thyroxine (Sigma Aldrich, St. Louis, MO, USA), by an orogastric tube, at a dose of 50 µg/animal/day, diluted in 5 mL of distilled water according to previously established protocols (Serakides et al., 2004; Ribeiro et al., 2018a, 2018b) for the entire gestation period and for three days of lactation. The females of the control group received the same volume of distilled water, using an orogastric tube, during the entire experimental period. Three days after birth, three neonates were randomly selected from each mother to obtain osteoblasts. Euthanasia was performed by anesthetic overdose with ketamine (100 mg/kg) and xylazine (30 mg/kg) intraperitoneally. The mothers were euthanized by cardiac puncture, preceded by anesthesia with ketamine (40 mg/kg) and xylazine (10 mg/kg) intraperitoneally.
Confirmation of maternal hyperthyroidism
The functional thyroid status of the mothers was determined using plasma analysis of free T4. On the third day of lactation, when the osteoblasts were extracted from the neonates, the blood of the mothers was drawn with an anticoagulant (heparin), and the plasma was separated by centrifugation and stored at −20°C for the determination of free T4, which was performed using the chemiluminescence ELISA technique (sensitivity: 0.4 ng/dL) with commercial kits according to the manufacturer’s instructions (IMMULITE, Siemens Medical Solutions Diagnostics, Malvern, PA, USA).
The thyroids from neonates were fixed in 10% formaldehyde and later processed and embedded in paraffin. 3 μm-thick sections were stained using the hematoxylin-eosin technique. The height of the epithelium was measured in 20 follicles at four points equidistant from the follicle with the aid of the ImageJ 1.52 k program (National Institute of Health, USA).
Isolation and culture of osteoblasts
Isolation and culture of osteoblasts were performed according to a previously described protocol (Reis et al., 2015). After euthanizing the neonates, antisepsis of the head skin was performed with a solution of polvidone iodine and 70% alcohol. A line incision was made in the skin from the cervical region to the snout, exposing the calvaria. The calvaria was then removed, dissected, and washed with sterile 0.15 M phosphate buffered saline (PBS). Subsequently, the fragments were incubated with 0.5% trypsin (Gibco, Grand Island, NY, USA) for 15 min at 37°C and 5% CO2. Trypsin was discarded, and the fragments were incubated with 0.2% collagenase II (Sigma, St Louis, MO, USA) for 30 min at 37°C and 5% CO2. Treatment with collagenase II was repeated twice. The supernatant was collected and centrifuged for 5 min at 1400×g. The pellet was resuspended in T75 bottles with Dulbecco’s modified eagle medium (DMEM) low glucose (Gibco, USA), gentamicin (60 mg/L), penicillin (100 IU/mL), streptomycin (100 mg/mL), amphotericin B (25 mg/L), and 10% fetal bovine serum (Gibco, USA). The medium was changed twice a week.
After the third passage, osteoblasts were transferred, according to the type of assay, to 24-well plates (1 × 104 cells/well) or T25 bottle (25 × 104 cells/well), and the medium was replaced with osteogenic medium, composed of basal culture medium with ascorbic acid (50 µg/mL), β-glycerophosphate (10 mM), and dexamethasone (0.1 µM), and kept in an incubator at 37°C and 5% CO2. The sampling unit was composed of the cell pool of the three neonates from each mother, totaling six units per group for each of the assays described below.
At 7, 14, and 21 days, samples from each group were subjected to the MTT (3-(4,5-dimethylthiazol-2yl)-2,5-diphenyltetrazolium bromide) conversion test. The culture medium was removed and osteoblasts were washed twice with sterile 0.15 M PBS solution. Osteogenic medium (210 µL) was added to 170 µL of MTT (Invitrogen, Carlsbad, CA, USA) (5 mg/mL). The plates were incubated at 37°C and 5% CO2 for 2 h. 210 µL of SDS-10% HCl was added. 100 µL from each well was transferred to a 96-well plate and measured using a spectrophotometer at a wavelength of 595 nm.
Alkaline phosphatase activity by BCIP/NBT method
At 7, 14, and 21 days, the culture medium was removed and osteoblasts were washed twice with 0.15 M PBS solution. BCIP/NBT solution containing alkaline phosphatase buffer (1 mL), NBT (nitro-blue tetrazoliumchloride) (4.4 mL), and BCIP (5-bromo-4-chloro-3′-indolyl phosphate p-toluidine salt) (3.3 mL) was added to each well. The plates were incubated at 37°C and 5% CO2 for 2 h. After incubation, 210 µL of 10% SDS-HCl solution was added to each well and the plates were incubated for 12 h in an incubator at 37°C and 5% CO2. Subsequently, 100 µL from each well was transferred to 96-well plates and measured using a spectrophotometer at a wavelength of 595 nm.
Determination of the total area of mineralized matrix
At 21 days, the wells were washed twice with 0.15 M PBS solution. The cells were fixed with 4% paraformaldehyde for 24 h. The cell culture were washed with distilled water and stained by von Kossa. Forty fields per repetition were photographed at 10× magnification and the images were analyzed with ImageJ version 1.45 s software to measure the total area of the mineralized matrix. Data were coded prior to analysis so that the treatment group was not identified prior to completion of the analysis.
Evaluation of gene transcript expression by real-time RT-PCR
At 21 days of culture, quantitative evaluation of gene expression of Runx2, Bmp2, Fgfr1, collagen type I, osteocalcin, and osteopontin was performed by real-time RT-PCR (RT-qPCR). Briefly, 1 µg of RNA was used for cDNA synthesis using the SuperScript III Platinum Two-Step qPCR kit with SYBR Green (Invitrogen). qRT-PCR was performed on a SmartCycler II thermal cycler (Cepheid, Carpinteria, CA, USA). The first amplification step of qRT-PCR was reverse transcription for 120 s at 50°C, followed by PCR with 45 cycles at 95°C for of 15 s and 60°C for 30 s. The fluorescence data were analyzed to obtain the CT values. Gene expression analyzed by the 2−ΔΔCT method, where sample values were calculated relative to the GAPDH Ct values. To assess the linearity and efficiency of qPCR amplification, standard curves for all transcripts were generated using serial dilutions of cDNA, in addition to the evaluation of the melting curve of the amplification products. Primers were designed based on the Rattus norvergicus mRNA sequence (Table 1).
The design was entirely random, and for each variable, the mean and standard deviation (SD) were determined. The statistical assumptions of normality and homoscedasticity of variances were evaluated using the Shapiro–Wilk and Brown–Forsythe methods, respectively. Data that violated any of these assumptions were subjected to a logarithmic transformation. The data were subjected to Student’s t-test for comparison of means, assuming a 5% error rate, using the SigmaPlot computer package.
Confirmation of maternal hyperthyroidism
Maternal hyperthyroidism was confirmed by the significant increase in plasma concentrations of free T4 in the treated group compared to that in the control group. In addition, hyperthyroid rats exhibited clinical signs characterized by hyperactivity and aggressiveness.
The thyroid follicles of newborn rats in the control group were covered by predominantly cuboidal epithelium and filled with dense and sometimes vacuolated colloid, while in newborns exposed to maternal hyperthyroidism, a predominance of follicles covered by flattened epithelium was observed, with a significant reduction in the height of the follicular epithelium when compared to the control group (Fig. 1).
Figure 1: (A) Plasma concentrations of free T4 (mean ± standard deviation) of maternal rats in the control and hyperthyroidism-induced groups *P < 0.05 (Student’s t test). (B) Thyroid follicular epithelium height (μm) (mean ± standard deviation) of control neonate rats and rats exposed to maternal hyperthyroidism *P < 0.05 (Student’s t test).
Maternal hyperthyroidism reduces the MTT conversion in the osteoblasts of the offspring
At 7 and 14 days, there was no significant difference between groups. At 21 days, osteoblasts from newborn rats exposed to maternal hyperthyroidism showed lower MTT conversion compared to the control group (Fig. 2).
Figure 2: (A) Photomicrograph of MTT reduction to formazan crystals. (B) Conversion of MTT to formazan crystals (mean ± standard deviation) in two-dimensional cultures of osteoblasts extracted from the calvaria of control neonate rats and rats exposed to maternal hyperthyroidism at 7, 14, and 21 days. *P < 0.05 (Student’s t test).
Maternal hyperthyroidism increases the alkaline phosphatase activity in the osteoblasts of the offspring
Alkaline phosphatase activity did not differ significantly between the groups after 7 days. However, at 14 and 21 days, osteoblasts extracted from newborn rats exposed to maternal hyperthyroidism showed significantly higher alkaline phosphatase activity than the control group (Fig. 3).
Figure 3: (A) Photomicrograph of alkaline phosphatase activity by the BCIP/NBT method. (B) Alkaline phosphatase activity (mean ± standard deviation) in two-dimensional cultures of osteoblasts extracted from the calvaria of control neonate rats and rats exposed to maternal hyperthyroidism at 7, 14, and 21 days. *P < 0.05 (Student’s t test).
Maternal hyperthyroidism increases the matrix synthesis of osteoblasts of the offspring
At 21 days, cultures of osteoblasts from newborn rats exposed to maternal hyperthyroidism presented a significantly larger total area of mineralized matrix compared to the control group (Fig. 4).
Figure 4: (A) Photomicrograph of culture of osteoblasts extracted from the calvaria of control neonate rats and rats exposed to maternal hyperthyroidism stained with von Kossa with increased total area of mineralized matrix at 21 days. (B) Total mineralized matrix area of osteoblasts extracted from the calvaria of control neonate rats and rats exposed to maternal hyperthyroidism at 21 days. *P < 0.05 (Student’s t test).
Maternal hyperthyroidism increases the expression of osteocalcin and osteopontin in the osteoblasts of the offspring
At 21 days, cultures of osteoblasts extracted from newborn rats exposed to maternal hyperthyroidism showed a significant increase in the gene expression of osteocalcin and osteopontin compared to the control group. There was no significant difference between the groups regarding the expression of Runx2, Bmp2, Fgfr1, and type I collagen (Fig. 5).
Figure 5: Gene transcript expression (mean ± standard deviation) of Runx2, Bmp2, Fgfr1, collagen type I, osteocalcin, and osteopontin by real-time quantitative polymerase chain reaction (RT-qPCR) technique in osteoblasts extracted from the calvaria of control neonate rats and rats exposed to maternal hyperthyroidism at 21 days. *P < 0.05 (Student’s t test).
In the current study, oral administration of L-thyroxine to adult female rats during the gestation period induced hyperthyroidism, as demonstrated by the significant increase in free T4. This result coincides with those of other studies, which were also successful in inducing hyperthyroidism during pregnancy (Silva et al., 2014; Maia et al., 2016; Ribeiro et al., 2018a, 2018b). Clinical signs also reinforced the success in inducing hyperthyroidism, since hyperactivity is an important clinical manifestation of hyperthyroidism in humans (Ahmed et al., 2008), and aggressiveness has already been reported in rats with induced hyperthyroidism (Silva et al., 2014; Ribeiro et al., 2018a, 2018b).
It is known that the plasma level of the mother’s thyroid hormones during gestation and lactation directly affects the thyroid function of the offspring, since the thyroid hormones are able to cross the placenta and reach the fetal circulation (Ahmed et al., 2008). The elevation of thyroid hormones in rats with induced hyperthyroidism is accompanied by elevated thyroid hormone levels in the offspring during the first three weeks of birth (El-bakry et al., 2010). In addition, maternal hyperthyroidism can alter the thyroid architecture of the offspring by decreasing the height of the epithelium lining. These changes become more pronounced with advancing age from day 1 to day 21 in postnatal pups (Ahmed et al., 2010). Thus, the decrease in the height of the epithelium of thyroid follicles in neonates at 3 days of age confirms the influence of maternal hyperthyroidism on the offspring.
The results of the present study demonstrate that maternal hyperthyroidism increases the matrix synthesis activity of the osteoblasts extracted from the calvaria of the offspring, as there was a greater total area of mineralized matrix, increased alkaline phosphatase activity, and increased gene expression of osteocalcin and osteopontin.
Alkaline phosphatase, an important marker of osteogenic differentiation, is one of the first enzymes expressed during mineralization. Although the mechanisms by which this enzyme exerts its functions are not well established, alkaline phosphatase is believed to be responsible for increasing the local concentration of inorganic phosphate, a mineralization promoter, and decreasing the concentration of extracellular pyrophosphate, an inhibitor of mineralization (Golub and Boesze-Battaglia, 2007). In addition to being an important marker, Nakamura et al. (2020) demonstrated that alkaline phosphatase also functions as a regulator of osteogenic differentiation, both by controlling the extracellular concentration of inorganic phosphate and by regulating the expression of Runx2.
Our results demonstrated that greater the activity of alkaline phosphatase and the expression of osteocalcin and osteopontin, greater the total area of mineralized matrix in cultures of osteoblasts extracted from neonates exposed to maternal hyperthyroidism. These results corroborate the changes observed in vitro, which demonstrated that the addition of thyroid hormones increases the synthesis activity of osteoblasts (Ohishi et al., 1994; Varga et al., 1997; Gouveia et al., 2001; Asai et al., 2009). The expression of non-collagenic proteins, including osteocalcin and osteopontin, is essential for the maturation and mineralization of the matrix, which is characterized in vitro by the formation of mineralized nodules. Osteopontin is an important phosphoprotein that has calcium-binding domains and is directly related to matrix mineralization. Osteocalcin, in turn, promotes bone matrix mineralization by regulating the growth of hydroxyapatite crystals, and is considered an important marker of well-differentiated osteoblasts (Roach, 1994; Nefussi et al., 1997). Thus, the increased synthesis activity of osteoblasts may be one of the mechanisms responsible for accelerating intramembranous growth, with early disappearance of sutures and premature fusion of the skull bones resulting from maternal hyperthyroidism.
The conversion of MTT to formazan crystals was lower in osteoblasts extracted from the group exposed to maternal hyperthyroidism at 21 days. MTT (3-(4,5-dimethylthiazol-2-yl)-2,5-diphenyltetrazolium bromide) is a widely used colorimetric assay for evaluating cell proliferation, cytotoxicity, and viability, which is based on the reduction of MTT to formazan crystals by metabolically active cells (Stockert et al., 2012; Präbst et al., 2017). Wang et al. (2004) demonstrated that MTT values correspond to the number of viable chondrocytes. Similarly, other studies have used MTT to assess osteoblast proliferation (Xu et al., 2012; Liu et al., 2016, 2017). Varga et al. (1997) demonstrated that the addition of T3 to osteoblasts in vitro decreased proliferation and stimulated osteoblastic differentiation, with increased expression of genes such as OC and ALP. The authors suggest that the decrease in proliferation was a consequence of T3-induced differentiation in osteoblasts. Osteoblast proliferation is inversely related to differentiation. Increased expression of gene transcription factors associated with mineralization (ALP, OC, and OP) negatively regulates proliferation (Stein et al., 1990; Lian and Stein, 1992). Thus, it is postulated that the reduction in the conversion of MTT to formazan crystals in the group exposed to maternal hyperthyroidism was due to decreased proliferation, associated with increased differentiation, as evidenced by the increase in the total area of mineralized matrix and the expression of osteocalcin and osteopontin.
Considering that Runx2 is an essential transcription factor for osteogenic differentiation (Komori, 2020), the expression of Runx2 also is expected to increase in the group exposed to maternal hyperthyroidism. However, in our study, the addition of thyroxine to cultures of osteoblasts from rat calvaria resulted in increased alkaline phosphatase activity and osteocalcin expression without increasing Runx2 gene expression. Nevertheless, the expression of Runx2 in a period prior to an increase in phosphatase and osteocalcin should be assessed in order to fully assess the possible increase in Runx2 (Cray et al., 2013). Runx2 is expressed in response to the signaling of BMP2, which is also an important factor expressed during osteogenic differentiation and mineralization (Marie, 2008; Chen et al., 2012), but like Runx2, there was no significant difference between groups at 21 days with respect to BMP2 expression.
In our study, there was no significant difference in the expression of Fgfr1 between the groups. On the other hand, the addition of T3 to osteoblast cultures increases the expression of Fgfr1 and Fgf2 and enhances FGF/FGFR activation (Stevens et al., 2003). Under normal conditions, FGF/FGFR signaling induces osteoblast differentiation and activity and plays an important role in the regulation of intramembranous ossification in the skull, and its alteration is an important cause of craniosynostosis (Ornitz and Marie, 2002; Rice et al., 2003). Since osteogenic differentiation is well established, Fgfr1 is downregulated (Iseki et al., 1999). However, it would be interesting to investigate the expression of the Fgfr1 transcript in the sutures of neonates born to mothers with hyperthyroidism and to investigate the expression of the protein and not only the gene transcript.
Thus, it can be concluded that maternal hyperthyroidism increases matrix synthesis activity, alkaline phosphatase activity, and expression of gene transcripts for osteocalcin and osteopontin in osteoblasts extracted from calvaria of the offsprings, which may be one of the mechanisms of premature fusion of cranial sutures.
Availability of Data and Materials:The datasets generated during and/or analyzed during the current study are available from the corresponding author on reasonable request.
Author Contribution:The authors confirm contribution to the paper as follows: study conception and design: F.A., R.S., N.O.; data collection: F.A., B.B., I.C.S.F., D.M.A., A.R., J.F; analysis and interpretation of results: F.A., R.S, N.O.; draft manuscript preparation: F.A., R.S. All authors reviewed the results and approved the final version of the manuscript.
Ethics Approval:Research involving animals has complied with all the relevant national regulations and institutional policies for the care and use of animals. The research has been approved by the by the Animal Use Ethics Committee of the Universidade Federal de Minas Gerais, Belo Horizonte, Minas Gerais, Brasil (Protocol No. 156/2020).
Funding Statement:This work was supported by grants from the Conselho Nacional de Desenvolvimento Científico e Tecnológico (CNPq), Fundação de Amparo a Pesquisa de Minas Gerais (Fapemig), and Coordenação de Aperfeiçoamento de Pessoal de Nível Superior (Capes).
Conflicts of Interest:The authors declare that they have no conflicts of interest to report regarding the present study.
References
Ahmed OM, El-Gareib AW, El-bakry AM, Abd El-Tawab SM, Ahmed RG (2008). Thyroid hormones states and brain development interactions. International Journal of Developmental Neuroscience 26: 147–209. DOI 10.1016/j.ijdevneu.2007.09.011. [Google Scholar] [CrossRef]
Ahmed OM, Abd El-Tawab SM, Ahmed RG (2010). Effects of experimentally induced maternal hypothyroidism and hyperthyroidism on the development of rat offspring: I. The development of the thyroid hormones-neurotransmitters and adenosinergic system interactions. International Journal of Developmental Neuroscience 28: 437–454. DOI 10.1016/j.ijdevneu.2010.06.007. [Google Scholar] [CrossRef]
Andersen SL, Andersen S (2021). Hyperthyroidism in pregnancy: Evidence and hypothesis in fetal programming and development. Endocrine Connections 10: R77–R86. DOI 10.1530/EC-20-0518. [Google Scholar] [CrossRef]
Asai S, Cao X, Yamauchi M, Funahashi K, Ishiguro N, Kambe F (2009). Thyroid hormone non-genomically suppresses Src thereby stimulating osteocalcin expression in primary mouse calvarial osteoblasts. Biochemical and Biophysical Research Communications 387: 92–96. DOI 10.1016/j.bbrc.2009.06.131. [Google Scholar] [CrossRef]
Bassett JHD, O’Shea PJ, Sriskantharajah S, Rabier B, Boyde A et al. (2007). Thyroid hormone excess rather than thyrotropin deficiency induces osteoporosis in hyperthyroidism. Molecular Endocrinology 21: 1095–1107. DOI 10.1210/me.2007-0033. [Google Scholar] [CrossRef]
Brent GA (2000). Tissue-specific actions of thyroid hormone: Insights from animal models. Reviews in Endocrine and Metabolic Disorders 1: 27–33. DOI 10.1023/A:1010056202122. [Google Scholar] [CrossRef]
Carmichael SL, Ma C, Rasmussen SA, Cunningham ML, Browne ML, Dosiou C, Lammer EJ, Shaw GM (2015). Craniosynostosis and risk factors related to thyroid dysfunction. American Journal of Medical Genetics A 167: 701–707. DOI 10.1002/ajmg.a.36953. [Google Scholar] [CrossRef]
Chen G, Deng C, Li YP (2012). TGF-β and BMP signaling in osteoblast differentiation and bone formation. International Journal of Biological Sciences 8: 272–288. DOI 10.7150/ijbs.2929. [Google Scholar] [CrossRef]
Cray JJ, Khaksarfard K, Weinberg SM, Elsalanty M, Yu JC (2013). Effects of thyroxine exposure on osteogenesis in mouse calvarial pre-osteoblasts. PLoS One 8: 1–9. DOI 10.1371/journal.pone.0069067. [Google Scholar] [CrossRef]
El-bakry AM, El-Gareib AW, Ahmed RG (2010). Comparative study of the effects of experimentally induced hypothyroidism and hyperthyroidism in some brain regions in albino rats. International Journal of Developmental Neuroscience 28: 371–389. DOI 10.1016/j.ijdevneu.2010.04.003. [Google Scholar] [CrossRef]
Golub EE, Boesze-Battaglia K (2007). The role of alkaline phosphatase in mineralization. Current Opinion in Orthopaedics 18: 444–448. DOI 10.1097/BCO.0b013e3282630851. [Google Scholar] [CrossRef]
Gouveia CH, Schultz JJ, Bianco AC, Brent GA (2001). Thyroid hormone stimulation of osteocalcin gene expression in ROS 17/2.8 cells is mediated by transcriptional and post-transcriptional mechanisms. Journal of Endocrinology 170: 667–675. DOI 10.1677/joe.0.1700667. [Google Scholar] [CrossRef]
Gouveia CHA, Miranda-Rodrigues M, Martins GM, Neofiti-Papi B (2018). Thyroid hormone and skeletal development. In: Vitamins and Hormones, pp. 383–472. Academic Press, Cambridge, Massachusetts, EUA: Elsevier. [Google Scholar]
Howie RN, Durham EL, Black L, Bennfors G, Parsons TE, Elsalanty ME, Yu JC, Weinberg SM, Cray JJ (2016). Effects of in Utero thyroxine exposure on murine cranial suture growth. PLoS One 11: 1–19. DOI 10.1371/journal.pone.0167805. [Google Scholar] [CrossRef]
Iseki S, Wilkie AOM, Morriss-Kay GM (1999). Fgfr1 and Fgfr2 have distinct differentiation- and proliferation-related roles in the developing mouse skull vault. Development 126: 5611–5620. DOI 10.1242/dev.126.24.5611. [Google Scholar] [CrossRef]
Jin SW, Sim KB, Kim SD (2016). Development and growth of the normal cranial vault: An embryologic review. Journal of Korean Neurosurgical Society 59: 192–196. DOI 10.3340/jkns.2016.59.3.192. [Google Scholar] [CrossRef]
Johnsonbaugh RE, Bryan RN, Hierlwimmer UR, Georges LP (1978). Premature craniosynostosis: A common complication of juvenile thyrotoxicosis. The Journal of Pediatrics 93: 188–191. DOI 10.1016/S0022-3476(78)80493-4. [Google Scholar] [CrossRef]
Komori T (2020). Molecular mechanism of Runx2-Dependent bone development. Molecules and Cells 43: 168–175. DOI 10.14348/molcells.2019.0244. [Google Scholar] [CrossRef]
Krassas GE, Poppe K, Glinoer D (2010). Thyroid function and human reproductive health. Endocrine Reviews 31: 702–755. DOI 10.1210/er.2009-0041. [Google Scholar] [CrossRef]
Leitch VD, Bassett JHD, Williams GR (2020). Role of thyroid hormones in craniofacial development. Nature Reviews Endocrinology 16: 147–164. DOI 10.1038/s41574-019-0304-5. [Google Scholar] [CrossRef]
Lian JB, Stein GS (1992). Concepts of osteoblast growth and differentiation: Basis for modulation of bone cell development and tissue formation. Critical Reviews in Oral Biology and Medicine 3: 269–305. DOI 10.1177/10454411920030030501. [Google Scholar] [CrossRef]
de Lima MA, Oliveira LB, Paim N, Borges MF (1999). Congenital hyperthyroidism: Autopsy report. Revista Do Hospital Das Clínicas 54: 103–106. DOI 10.1590/S0041-87811999000300007. [Google Scholar] [CrossRef]
Liu X, Song X, Zhang P, Zhu Z, Xu X (2016). Effects of nano tantalum implants on inducing osteoblast proliferation and differentiation. Experimental and Therapeutic Medicine 12: 3541–3544. DOI 10.3892/etm.2016.3801. [Google Scholar] [CrossRef]
Liu M, Xu H, Ma Y, Cheng J, Hua Z, Huang G (2017). Osteoblasts proliferation and differentiation stimulating activities of the main components of Epimedii folium. Pharmacognosy Magazine 13: 90–94. DOI 10.4103/0973-1296.197654. [Google Scholar] [CrossRef]
Maia MZ, Santos GK, Batista ACM, Reis AMS, Silva JF, Ribeiro LGR, Ocarino NDM, Serakides R (2016). Effects of excess maternal thyroxin on the bones of rat offspring from birth to the post-weaning period. Archives of Endocrinology and Metabolism 60: 130–137. DOI 10.1590/2359-3997000000168. [Google Scholar] [CrossRef]
Marie PJ (2008). Transcription factors controlling osteoblastogenesis. Archives of Biochemistry and Biophysics 473: 98–105. DOI 10.1016/j.abb.2008.02.030. [Google Scholar] [CrossRef]
Nakamura T, Nakamura-Takahashi A, Kasahara M, Yamaguchi A, Azuma T (2020). Tissue-nonspecific alkaline phosphatase promotes the osteogenic differentiation of osteoprogenitor cells. Biochemical and Biophysical Research Communications 524: 702–709. DOI 10.1016/j.bbrc.2020.01.136. [Google Scholar] [CrossRef]
Neale DM, Cootauco AC, Burrow G (2007). Thyroid disease in pregnancy. Clinics in Perinatology 34: 543–557. DOI 10.1016/j.clp.2007.10.003. [Google Scholar] [CrossRef]
Nefussi JR, Brami G, Modrowski D, Obœuf M, Forest N (1997). Sequential expression of bone matrix proteins during rat calvaria osteoblast differentiation and bone nodule formation in vitro. Journal of Histochemistry and Cytochemistry 45: 493–503. DOI 10.1177/002215549704500402. [Google Scholar] [CrossRef]
O’Shea PJ, Harvey CB, Suzuki H, Kaneshige M, Kaneshige K, Cheng SY, Williams GR (2003). A thyrotoxic skeletal phenotype of advanced bone formation in mice with resistance to thyroid hormone. Molecular Endocrinology 17: 1410–1424. DOI 10.1210/me.2002-0296. [Google Scholar] [CrossRef]
O’Shea PJ, Bassett JHD, Sriskantharajah S, Ying H, Cheng SY, Williams GR (2005). Contrasting skeletal phenotypes in mice with an identical mutation targeted to thyroid hormone receptor α1 or β. Molecular Endocrinology 19: 3045–3059. DOI 10.1210/me.2005-0224. [Google Scholar] [CrossRef]
Ohishi K, Ishida H, Nagata T, Yamauchi N, Tsurumi C, Nishikawa S, Wakano Y (1994). Thyroid hormone suppresses the differentiation of osteoprogenitor cells to osteoblasts, but enhances functional activities of mature osteoblasts in cultured rat calvaria cells. Journal of Cellular Physiology 161: 544–552. DOI 10.1002/(ISSN)1097-4652. [Google Scholar] [CrossRef]
Opperman LA (2000). Cranial sutures as intramembranous bone growth sites. Developmental Dynamics 219: 472–485. DOI 10.1002/(ISSN)1097-0177. [Google Scholar] [CrossRef]
Ornitz DM, Marie PJ (2002). FGF signaling pathways in endochondral and intramembranous bone development and human genetic disease. Genes and Development 16: 1446–1465. DOI 10.1101/gad.990702. [Google Scholar] [CrossRef]
Präbst K, Engelhardt H, Ringgeler S, Hübner H (2017). Basic colorimetric proliferation assays: MTT, WST, and resazurin. Methods in Molecular Biology 1601: 1–17. DOI 10.1007/978-1-4939-6960-9. [Google Scholar] [CrossRef]
Rasmussen SA, Yazdy MM, Carmichael SL, Jamieson DJ, Canfield MA, Honein MA (2007). Thyroid disease as a risk factor for Craniosynostosis. Obstetrics & Gynecology 110: 369–377. DOI 10.1097/01.AOG.0000270157.88896.76. [Google Scholar] [CrossRef]
Reis AMS, Ribeiro LGR, De Melo Ocarino N, Goes AM, Serakides R (2015). Osteogenic potential of osteoblasts from neonatal rats born to mothers treated with caffeine throughout pregnancy. BMC Musculoskeletal Disorders 16: 1–11. DOI 10.1186/s12891-015-0467-8. [Google Scholar] [CrossRef]
Ribeiro LGR, Silva JF, Ocarino NDM, Melo EGD, Serakides R (2018a). Excess maternal and postnatal thyroxine alters chondrocyte numbers and the composition of the extracellular matrix of growth cartilage in rats. Connective Tissue Research 59: 73–84. DOI 10.1080/03008207.2017.1290084. [Google Scholar] [CrossRef]
Ribeiro LGR, Silva JF, Ocarino NDM, Souza CAD, Melo EGD, Serakides R (2018b). Excess maternal thyroxine alters the proliferative activity and angiogenic profile of growth cartilage of rats at birth and weaning. Cartilage 9: 89–103. DOI 10.1177/1947603516684587. [Google Scholar] [CrossRef]
Rice DPC, Rice R, Thesleff I (2003). Fgfr mRNA isoforms in craniofacial bone development. Bone 33: 14–27. DOI 10.1016/S8756-3282(03)00163-7. [Google Scholar] [CrossRef]
Roach HI (1994). Why does bone matrix contain non-collagenous proteins? The possible roles of osteocalcin, osteonectin, osteopontin and bone sialoprotein in bone mineralisation and resorption. Cell Biology International 18: 617–628. DOI 10.1006/cbir.1994.1088. [Google Scholar] [CrossRef]
Segni M, Leonardi E, Mazzoncini B, Pucarelli I, Pasquino AM (1999). Special features of Graves’ disease in early childhood. Thyroid 9: 871–877. DOI 10.1089/thy.1999.9.871. [Google Scholar] [CrossRef]
Serakides R, Nunes VA, de Ocarino NM, Nascimento EFD (2004). Efeito da Associação Hipertireoidismo—Castração no Osso de ratas adultas. Arquivo Brasileiro de Endocrinologia e Metabologia 48: 875–884. DOI 10.1590/S0004-27302004000600015. [Google Scholar] [CrossRef]
Silva JF, Ocarino NM, Serakides R (2014). Maternal thyroid dysfunction affects placental profile of inflammatory mediators and the intrauterine trophoblast migration kinetics. Reproduction 147: 803–816. DOI 10.1530/REP-13-0374. [Google Scholar] [CrossRef]
Springer D, Jiskra J, Limanova Z, Zima T, Potlukova E (2017). Thyroid in pregnancy: From physiology to screening. Critical Reviews in Clinical Laboratory Sciences 54: 102–116. DOI 10.1080/10408363.2016.1269309. [Google Scholar] [CrossRef]
Stein GS, Lian JB, Owen TA (1990). Bone cell differentiation: A functionally coupled relationship between expression of cell-growth-and tissue-specific genes. Current Opinion in Cell Biology 2: 1018–1027. DOI 10.1016/0955-0674(90)90151-4. [Google Scholar] [CrossRef]
Stevens DA, Harvey CB, Scott AJ, O’Shea PJ, Barnard JC, Williams AJ, Brady G, Samarut J, Chassande O, Williams GR (2003). Thyroid hormone activates fibroblast growth factor receptor-1 in bone. Molecular Endocrinology 17: 1751–1766. DOI 10.1210/me.2003-0137. [Google Scholar] [CrossRef]
Stockert JC, Blázquez-Castro A, Cañete M, Horobin RW, Villanueva Á. (2012). MTT assay for cell viability: Intracellular localization of the formazan product is in lipid droplets. Acta Histochemica 114: 785–796. DOI 10.1016/j.acthis.2012.01.006. [Google Scholar] [CrossRef]
Taylor PN, Albrecht D, Scholz A, Gutierrez-buey G, Lazarus JH, Dayan CM, Okosieme OE (2018). Global epidemiology of hyperthyroidism and hypothyroidism. Nature 14: 301–316. DOI 10.1038/nrendo.2018.18. [Google Scholar] [CrossRef]
Twigg SRF, Wilkie AOM (2015). A genetic-pathophysiological framework for craniosynostosis. American Journal of Human Genetics 97: 359–377. DOI 10.1016/j.ajhg.2015.07.006. [Google Scholar] [CrossRef]
Varga F, Rumpler M, Luegmayr E, Fratzl-Zelman N, Glantschnig H, Klaushofer K (1997). Triiodothyronine, a regulator of osteoblastic differentiation: Depression of histone H4, attenuation of c-fos/c-jun, and induction of osteocalcin expression. Calcified Tissue International 61: 404–411. DOI 10.1007/s002239900356. [Google Scholar] [CrossRef]
Wang G, Woods A, Sabari S, Pagnotta L, Stanton LA, Beier F (2004). RhoA/ROCK signaling suppresses hypertrophic chondrocyte differentiation. Journal of Biological Chemistry 279: 13205–13214. DOI 10.1074/jbc.M311427200. [Google Scholar] [CrossRef]
Xu W, Xu L, Chen M, Mao YT, Xie ZG, Wu SL, Dong QR (2012). The effects of low dose X-irradiation on osteoblastic MC3T3-E1 cells in vitro. BMC Musculoskeletal Disorders 13: 94. DOI 10.1186/1471-2474-13-94. [Google Scholar] [CrossRef]
Cite This Article
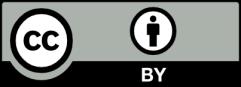
This work is licensed under a Creative Commons Attribution 4.0 International License , which permits unrestricted use, distribution, and reproduction in any medium, provided the original work is properly cited.