Open Access
REVIEW
High density lipoprotein as a therapeutic target: Focus on its functionality
School of Pharmacy and Biochemistry, Laboratory of Lipids and Atherosclerosis, Department of Clinical Biochemistry, University of Buenos Aires, National Scientific and Technical Research Council (CONICET), Buenos Aires, 1113, Argentina
* Corresponding Author: MAXIMILIANO MARTíN. Email:
(This article belongs to the Special Issue: Genetic Polymorphism and Metabolic inflammation in Development of Insulin Resistance and Metabolic Syndrome)
BIOCELL 2023, 47(11), 2361-2383. https://doi.org/10.32604/biocell.2023.031063
Received 11 May 2023; Accepted 22 September 2023; Issue published 27 November 2023
Abstract
Cardiovascular diseases (CVDs) are the leading cause of death globally. CVDs are a group of disorders of the heart and blood vessels and include coronary heart disease, cerebrovascular disease and rheumatic heart disease among other conditions. There are multiple independent risk factors for CVD, including hypertension, age, smoking, insulin resistance, elevated low-density lipoprotein cholesterol (LDL-C) levels, and triglyceride levels. LDL-C levels have traditionally been the target for therapies aimed at reducing CVD risk. High density lipoprotein (HDL) constitutes the only lipoprotein fraction with atheroprotective functions. Early HDL-targeted therapies have focused on increasing HDL-C levels. However, clinical trials have shown that raising HDL-C with niacin failed to achieve CVD reduction. A possible explanation for these findings is that these drugs could interfere with lipid metabolism and cause alterations in HDL structure and composition, leading to loss of functionality. As a result, targeting HDL-C levels would be insufficient to achieve CVD risk reduction, making HDL functionality a more desirable focus for HDL-directed therapies. There are several drugs which show the potential to improve HDL functionality. These drugs include molecules already approved for human use, such as statins and niacin, and particularly, compounds currently undergoing development such as apolipoprotein A-I mimetics and reconstituted HDL preparations. These therapies show promising potential to improve HDL functionality specifically. Future therapeutic strategies should incorporate HDL functionality as a main target of interest.Graphic Abstract
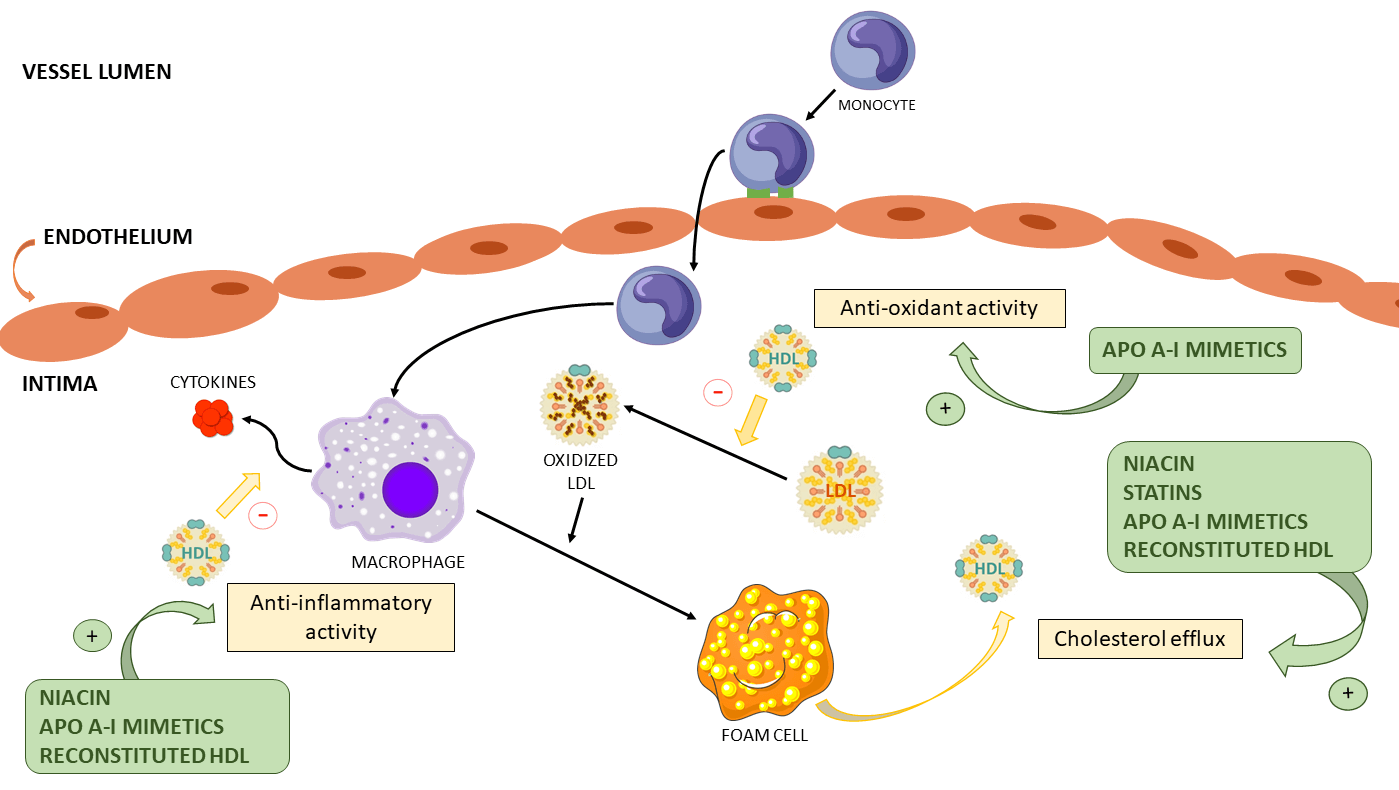
Keywords
Cardiovascular diseases (CVDs) stand as the primary global cause of mortality, accounting for 17.9 million fatalities annually. CVDs encompass a range of heart and blood vessel disorders, including coronary heart disease, cerebrovascular disease, and rheumatic heart disease, among other conditions (Cardiovascular Diseases). Numerous distinct risk factors contribute to the development of CVD, such as hypertension, age, smoking, insulin resistance, elevated concentration of low-density lipoprotein cholesterol (LDL-C), and triglyceride levels (Linton et al., 2019; Huang et al., 2020). In the majority of individuals, atherosclerotic plaques start to form during young adulthood, emphasizing the importance of focusing on plaque regression as the preferred therapeutic strategy (Huang et al., 2020). LDL-C levels have traditionally been the target for therapies aimed at reducing CVD risk (Karalis, 2009). However, despite 78 weeks of treatment, the most potent LDL-lowering agent, the proprotein convertase subtilisin/kexin type 9 (PCSK9) inhibitor evolocumab, only resulted in a modest regression of coronary atheroma volume, as measured through serial coronary intravascular ultrasound, with a decrease of just 0.95%, despite lowering LDL-C levels by 60% (Nicholls et al., 2016; Puri et al., 2016).
For quite some time, it has been established that decreased levels of high density lipoprotein cholesterol (HDL-C) in the bloodstream is associated with increased risk of atherosclerotic CVD (Miller and Miller, 1975; Gordon et al., 1977). Remarkably, HDL constitutes the only lipoprotein fraction with atheroprotective functions (Navab et al., 2011). These functions include its ability to promote reverse cholesterol transport (RCT) and its antioxidant, antiinflammatory and antiapoptotic activities, among others (Navab et al., 2011). Traditionally, HDL particles have been evaluated in the clinical setting by measuring their cholesterol content and the concentration of their main apolipoprotein (apo), apo A-I (Walldius and Jungner, 2007). In this context, it is worth discussing the role of cholesteryl ester transfer protein (CETP) and lecithin:cholesterol acyltransferase (LCAT). CETP plays a crucial role in HDL metabolism by facilitating the transfer of cholesteryl esters from HDL to apolipoprotein (apo) B-containing lipoproteins in exchange for TG. Thus, CETP deficiency has been associated with an increase in HDL particle size and HDL-C content, while CETP overexpression has been linked to smaller HDL particles and a decrease in HDL-C levels (Chapman et al., 2010). On the other hand, LCAT is an enzyme whose function is to convert free cholesterol to esterified cholesterol, increasing its hydrophobicity. As a result, cholesterol is sequestered towards the core of the lipoprotein particle, causing it to acquire a spherical shape (Dobiásová and Frohlich, 1999). Deficiency in the activity of this enzyme is associated with low levels of HDL-cholesterol (HDL-C) (Calabresi et al., 2012). However, there is controversy regarding the association between this deficiency and an increased risk of atherosclerosis (Savel et al., 2012).
Early HDL-targeted therapies have focused on increasing HDL-C levels. Indeed, significant strides have been taken to increase plasma HDL-C levels with medications like niacin and CETP inhibitors (Huang et al., 2020; Mani and Rohatgi, 2015; Tall and Rader, 2017). Nevertheless, clinical trials have demonstrated that raising HDL-C levels with niacin did not result in a reduction in CVD (Mani and Rohatgi, 2015; Hung et al., 2019). Similar findings have been observed with CETP inhibitors (Tall and Rader, 2017; Bowman et al., 2017). One plausible explanation for these outcomes is that these drugs might affect lipid metabolism, inducing changes in the structure and composition of HDL, ultimately leading to a loss of its functional properties (Marsche, 2015). It is worth noting that plasma HDL particles exhibit significant heterogeneity in terms of their structure, size, composition, and biological roles, that may explain why measures of HDL-C and cardiovascular risk do not always correlate well. HDL can be separated by sequential ultracentrifugation on the basis of density, resulting in the large buoyant HDL2 and the small dense HDL3, which can be further subfractionated into 5 distinct subpopulations (HDL2b, HDL2a, HDL3a, HDL3b and HDL3c) (Camont et al., 2011). HDL subpopulations interact with different cellular receptors to remove excess cholesterol from cells, a key pathway by which HDL may contribute to atheroprotection (Camont et al., 2011). In fact, elevating HDL-C levels through pharmaceutical means using niacin and CETP inhibitors can potentially impact HDL subpopulations, resulting in elevated cholesterol levels within HDLs and an enlargement of HDL particles (Zanoni et al., 2016; Timón-Zapata et al., 2011; Madsen et al., 2018). When compared to the smaller, denser HDL3 particles, these larger HDL subtypes may not interact as effectively with ATP-binding cassette transporter A1 (ABCA1) and ABCG (Marsche, 2015). The interaction between HDL with ABCA1 and ABCG1 is crucial in facilitating the process of cholesterol efflux from macrophages to HDLs, which represents the initial step of RCT. Moreover, CETP inhibition could increase insulin resistance and cause fatty liver in CETP expressing transgenic mice fed with a high-fat diet (Zhu et al., 2018). Importantly, there is compelling evidence indicating that the capacity of HDL to promote cholesterol efflux is inversely correlated with CV events, even after adjusting for other risk factors, such as HDL-C and apo A-I levels (Rohatgi et al., 2014; Ebtehaj et al., 2019). Nevertheless, it remains challenging to determine, considering both clinical and biological perspectives, whether certain subfractions exhibit superior in vivo antiatherogenic properties than others. This may be attributed to the fact that the antiatherogenic functions exerted by HDL are based on dynamic processes, and static assessment of isolated particles would not reflect the coordinated work that different subfractions exert in vivo. As a result, targeting HDL-C levels would be insufficient to achieve CVD risk reduction, making the incorporation of total HDL functionality a desirable outcome for HDL-directed therapies.
HDL is a circulating heterogenic complex of about 50% lipids and 50% proteins, which in circulation carries different enzymes and lipid transport proteins. In fact, proteomic and lipidomic studies have identified hundreds of individual proteins and more than 200 lipid species in these particles (Kontush et al., 2013; Davidson et al., 2022).
Regarding HDL lipidome, it is well known that HDL particles carry free cholesterol, cholesteryl esters and triglycerides (Kontush et al., 2013). In addition, they also contain numerous lipid species such as sphingomyelin, ceramide, phosphatidylcholine, lysophosphatidylcholine, phosphatidic acid, phosphatidyl serine, and sphingosine-1 phosphate, among others (Kontush et al., 2013; Brites et al., 2017).
In terms of HDL proteome, apo A-I and apo A-II constitute the two most abundant apolipoproteins present in HDL particles (Davidson et al., 2022). Beyond them, HDLs also contain apo A-IV, apo C-II, apo C-III, apo C-IV, apo D, apo E, apo F, apo H, apo J, apo L-1 and apo M (Brites et al., 2017; Heinecke, 2009). Moreover, in plasma, HDL is associated to enzymes and lipid transport proteins responsible for its metabolism such as LCAT, phospholipid transfer protein (PLTP) and CETP (Brites et al., 2017). In addition, HDL particles can become enriched in proinflammatory and prooxidant markers, such as serum amyloid A (SAA) 1, SAA 2, SAA 4 and myeloperoxidase (Heinecke, 2009). In contrast HDL carries several antioxidant enzymes like paraoxonase (PON) 1, PON 3, glutathione peroxidase 3 and lipoprotein-associated phospholipase A2 (Brites et al., 2017). Finally, there are additional proteins associated with HDL (more than 200), that are relatively scarce and often only weakly bound to HDL particles. Despite their low abundance, these auxiliary proteins may play crucial roles in different biological processes (Davidson et al., 2022; Goetze et al., 2021).
The presence and interaction of these lipids, proteins and enzymes determine HDL atheroprotective capacity, highlighting the importance of HDL individual components.
HDL particles present multiple atheroprotective activities. Among these, the two most studied are their capability to facilitate RCT and to inhibit the oxidation of LDL particles. (Brites et al., 2017; Toth, 2003). As previously mentioned, RCT involves multiple steps, with the first being the promotion of cholesterol efflux from cells to HDL particles (Martin et al., 2022). The second and third steps are carried out by LCAT and CETP, respectively (Martin et al., 2022). However, it should be noted that HDL has other properties such as its antiinflammatory, antithrombotic and antiapoptotic activities. In particular, HDL suppresses the expression of adhesion molecules on endothelial cells, consequently reducing the recruitment of blood monocytes to the arterial wall, in addition to inhibiting cytokine expression on these inflammatory cells (Barter et al., 2004). HDL also increases nitric oxide (NO) production through upregulation of endothelial NO synthase (eNOS) expression. In fact, HDL stimulates eNOS through activation of the kinase cascade by HDL scavenger class B receptor type 1 (SRB1) (Mineo et al., 2006; Kosmas et al., 2018). HDL’s antithrombotic role can be manifested by stimulating prostacyclin synthesis, as well as by reducing tissue factor and selectin expression, resulting in the subsequent suppression of thrombin generation via the protein C pathway, inhibiting platelet activation (Mineo et al., 2006; Kosmas et al., 2018). Furthermore, the potential cytoprotective impact of HDL on endothelial cells may involve the inhibition of the apoptotic pathway responsible for endothelial cell self-destruction, achieved through a reduction in the activity of P32-like proteases (Kosmas et al., 2018; Sugano et al., 2000). Fig. 1 shows the main cardioprotective functions of HDL.
Figure 1: HDL multiple atheroprotective activities. 1) Inhibits the expression of adhesion molecules on endothelial cells and thus decreases the recruitment of blood monocytes to the arterial wall. 2) Antithrombotic function: Inhibits platelet activation by activating prostacyclin synthesis and attenuating tissue factor and selectin expression, regulating negatively thrombin generation through the protein C pathway. 3) Antiapoptotic function: prevention of the suicide pathway on endothelial cells. 4) Anti-inflammatory function: Inhibits cytokine expression. 5) Anti-oxidant function: prevents the oxidation of LDL particles. 6) Promotes RCT by promoting cholesterol efflux from cells to HDL particles. HDL, high density lipoprotein; LDL, low density lipoprotein.
Alterations in HDL functionality in different pathologies
Different pathologies can affect HDL quality and functionality. In particular, dysfunctional HDL particles are frequently found in pathologies characterized by the presence of inflammation and oxidative stress (Bonizzi et al., 2021). Our group has described alterations of HDL functionality in multiple pathologies. In a prior study we reported impaired capacity to promote cholesterol efflux in HDL from patients with metabolic diseases such as hypertriglyceridemia, obesity and type 2 diabetes (Martin et al., 2022; Brites et al., 1999, 2000). Furthermore, we also reported impaired cholesterol efflux in pathologies that affect iron metabolism like iron deficiency and iron overload (Meroño et al., 2015, 2017). Moreover, we described diminished cholesterol efflux in an autoimmune pathology such as rheumatoid arthritis (Pierini et al., 2021). In addition to alterations in reverse cholesterol transport, our group also reported impaired HDL antioxidant activity in a wide array of pathologies such as metabolic syndrome, type 2 diabetes, obesity, iron deficiency, iron overload and rheumatoid arthritis (Martin et al., 2022; Gomez Rosso et al., 2017, 2014; Gómez Rosso et al., 2008; Meroño et al., 2011; Meroño et al., 2010). Furthermore, other authors have described alterations in HDL functionality in pathologies such as cancer (Ganjali et al., 2019), endocrine disorders (van der Boom et al., 2020) and autoimmune diseases (Sánchez-Pérez et al., 2020).
Evaluation of HDL functionality
The complexities of HDL metabolism offer a huge challenge that researchers must face and that are relevant to the understanding of human biology to transform them into clinical tools. To assess the issue of HDL function as a potential therapeutic target, robust and simple analytical methods are required for its evaluation. In this regard, the complex pleiotropic effects of HDL make the development of a single measurement challenging. Laboratory assays that accurately measure HDL functionality must be developed, validated and brought to high-throughput for clinical purposes (Hafiane and Genest, 2015; Sacks and Jensen, 2018). Furthermore, HDL particles present multiple atheroprotective functions, so developing a single measure that can account for all of HDL properties makes the challenge even greater (Hafiane and Genest, 2015). A major confounding factor that makes the development of standardized assays difficult is the method by which HDL particles are isolated (Hafiane and Genest, 2015). Indeed, HDL can be isolated by selective precipitation, ultracentrifugation and electrophoresis, among other methods (Hafiane and Genest, 2015). These techniques can influence the results observed. Cholesterol efflux has received much attention as a biomarker that could reflect HDL functionality in vivo (Sacks and Jensen, 2018). Nevertheless, it remains unclear whether therapies directed at increasing cholesterol efflux can be atheroprotective (Ronsein and Heinecke, 2017). Furthermore, even though increasing HDL-C levels has shown little benefit to CVD, there is no doubt about the inverse association between HDL-C and CVD. However, the benefit of HDL-C appears to plateau at 61 mg/dL (Pownall and Gotto, 2019; di Angelantonio et al., 2009). In our opinion, the preferable approach to HDL-directed therapies should seek to achieve a balance between classic measures of HDL status such as HDL-C and new measures that better reflect HDL quality.
HDL Functionality and Pharmacological Therapy
There are several drugs in use or in development which show the potential to improve HDL functionality in conditions that show impairment of these atheroprotective functions. These therapies include, niacin, CETP and PCSK9 inhibitors, recombinant LCAT, statins, pioglitazone, metformin, ezetimibe, fibrates, lomitapide, RNA based drugs, apo A-I mimetics and rHDL (Niacin-Health Professional, 2022; Wu and Zhao, 2009; Zhang et al., 2017; Ward et al., 2019; Muñoz-Hernandez et al., 2018; Durairaj et al., 2017; Weinreich and Frishman, 2014; Smith, 2001; Rena et al., 2017; Islam et al., 2018; Darabi et al., 2016). Therefore, these drugs which have been shown to be capable of affecting HDL functionality will be the main subject of the present review.
Niacin is available in two forms, oral extended-release tablets (ERT) and immediate-release tablets (IRT). Administration of 1 to 3 grams per day of ERT has been shown to lower serum LDL-C levels while elevating HDL-C levels. Fig. 2 shows niacin proposed mechanism of action. IRT are available in formulations ranging from 50 to 500 mg. It is advisable to initiate therapy with the lowest feasible dosage to minimize the occurrence of adverse reactions (Djadjo and Bajaj, 2023). Niacin adverse effects include severe skin flushing, tachycardia, palpitations, diarrhea and nausea (Habibe and Kellar, 2023). Niacin can also present adverse interactions with alcohol, antidiabetic drugs, and statins among others (Habibe and Kellar, 2023). Evidence suggests that several genetic markers associated with levels of traditional lipid parameters such as TG, LDL-C and HDL-C can influence the response to niacin treatment (Tuteja et al., 2018). Niacin originally received approval from the US Food and Drug Administration (FDA) for the purpose of secondary prevention of CVD (Kamanna et al., 2009). Over time, its approved indications were expanded to include managing primary hyperlipidemia and mixed dyslipidemia, reducing triglyceride levels, and preventing CV events when combined with statins (D’Andrea et al., 2019). However, the FDA has recently announced withdrawal of previously approved indications for the use of extended-release niacin co-administered with a statin, based on evidence showing that reducing triglycerides or raising HDL-C with any drug does not improve CV risk in patients on statins (Ronsein et al., 2021). In accordance, a recent study quantifying the HDL proteome of subjects in 2 niacin clinical trials, the CPC study (Carotid Plaque Composition) and the HDL Proteomics analysis of the AIM-HIGH trial (Atherothrombosis Intervention in Metabolic Syndrome with Low HDL/High Triglycerides), showed that adding niacin to statin therapy increases the abundance of auxiliary proteins linked to atherogenesis, suggesting a possible explanation why niacin therapy fails to provide cardioprotection (Masana et al., 2015).
Figure 2: Hypothetical hypolipidemic mechanisms of niacin. Niacin inhibits adenylyl cyclase, resulting in decreased activation of protein kinase A (PKA) and cAMP levels, which together lead to reduced lipolysis. As a result, free fatty acid (FFA) plasma levels drop, and triglyceride (TG) synthesis and apolipoprotein C3 (apo C3) expression in the liver are reduced, leading to decreased very low-density lipoprotein (VLDL) formation. In addition, in vitro studies suggest that niacin also reduces TG synthesis and VLDL secretion by a direct inhibitory effect on diacylglycerol acyltransferase 2 (DGAT2). HSL, hormone sensitive lipase; ATGL, adipose TG lipase.
Regarding HDL function, pharmacological treatment with niacin reduced CETP and LCAT mass and activity in type 2 diabetic patients. In addition, HDL antioxidant associated enzymes, PON1 and Lp-PLA2, were signicantly decreased. However, niacin treatment was not associated with modifications of the oxidative process according to the lipoperoxide concentration in apo B-depleted plasma, which is an indirect index of HDL oxidation, and the capability of HDL to protect LDL against oxidation (Yvan-Charvet et al., 2010). In addition, another study showed that niacin treatment increased HDL cholesterol efflux from macrophage foam cells and inhibited inflammatory responses induced by toll-like receptor 4 (Yvan-Charvet et al., 2010; Lukasova et al., 2011). Finally, a mouse model study has demonstrated that, in response to niacin, the G-protein coupled receptor, hydroxycarboxylic acid receptor-2 (HCAR2) on monocytoid cells and macrophages leads to suppression of inflammatory responses and increased cholesterol efflux (Lukasova et al., 2011). This evidence suggests a new potential application for niacin in pharmacology.
In the bloodstream, CETP predominantly associates with HDL and plays a key role in facilitating the transfer of cholesteryl ester and triglycerides between HDL particles and apolipoprotein B-containing lipoproteins, as well as among different subtypes of the latter (Barter et al., 2003). Studies using pharmacological CETP inhibitors have shown promising results in animal models, with reductions in atherosclerotic plaque burden when CETP activity was inhibited (Nelson et al., 2022). Nevertheless, different clinical trials using orally taken pharmacological CETP inhibitors torcetrapib, dalcetrapib, evacetrapib, and anacetrapib have shown that the risk of CVD did not decrease even when substantial increases in HDL-C levels were achieved (Barter et al., 2007; Nicholls et al., 2017; Lincoff et al., 2017; Schwartz et al., 2012). Furthermore, some CETP inhibitors showed adverse effects such as increased blood pressure, inflammation and mortality (Taheri et al., 2020; Tardif et al., 2017). Genetic polymorphisms are one of the factors that could influence individual response to CETP inhibition and contribute to explain the results discussed above. In this regard, a pharmacogenomics study reported that dalcetrapib could lead to a significant reduction of cardiovascular events only in the carriers of the AA genotype for the rs1967309 single-nucleotide polymorphism of adenylate cyclase 9 (Tardif et al., 2015). However, these results could not be confirmed in a later study carried out in patients who presented acute coronary syndrome (Tardif et al., 2022).
Pharmacological CETP inhibition may result in the dysfunction of HDL by extending the half-life of HDL particles. This can, in turn, make them more vulnerable to adverse changes in their lipid and protein composition, as well as oxidative and enzymatic modifications of their protein and/or lipid components (von Eckardstein, 2022). However, different studies in human patients showed that cholesterol efflux was rather increased upon treatment with CETP inhibitors (Brodeur et al., 2017; Metzinger et al., 2020; Nicholls et al., 2015).
A recent work showed that the CETP inhibitors evacetrapib and torcetrapib increase apo A-I in HDL subspecies that also contain apo C-III (Furtado et al., 2022). The presence of apo C-III on HDL that also contain apo E eliminates its protective association against coronary heart disease by impairing metabolic pathways active in RCT that carries cholesterol from macrophages to the liver (Morton et al., 2018). By contrast, in another study including 377 dyslipidemic patients, evacetrapib monotherapy increased dose-dependent total and non–ABCA1-specific cell cholesterol efflux and also increased ABCA1-specific cell cholesterol efflux. Comparing with statin monotherapy, evacetrapib associated with statins also increased total, non–ABCA1- and ABCA1-specific cell cholesterol efflux (Nicholls et al., 2015), suggesting that cholesterol efflux capacity is substantially increased when potent CETP inhibitors are used alone vs. when they are added to statins.
These results reveal the complex effects of potent CETP inhibition on HDL remodeling and function that require further investigation.
A promising area of research is the inhibition of PCSK9, a protein that plays a key role in regulating cholesterol levels in the body. PCSK9 works by binding to LDL receptors (LDLr) on the surface of liver cells, causing the receptors to be internalized and degraded (Suchowerska et al., 2022). Fig. 3 shows PCSK9 mechanism of action. At present, there are two FDA-approved monoclonal antibodies that effectively inhibit the activity of PCSK9: alirocumab and evolocumab. Both can be administrated subcutaneously every two weeks or once monthly, depending on the dose (Tsouka et al., 2018). Recently, both the European Union and the FDA have granted approval for inclisiran, which is a small interfering mRNA designed to hinder the intracellular synthesis of PCSK9. The recommended dosing regimen involves an initial 284 mg subcutaneous injection, followed by another injection at 3-month, and subsequent injections every 6 months thereafter (Ray et al., 2023). The elevated cost of this particular treatment and the route of administration have a significant impact on the adherence of patients to this pharmacological alternative. The most common side effects of PCSK9 inhibitors are injection-site reactions and Influenza-like symptoms (Gürgöze et al., 2019). Pharmacogenomic studies carried out in individuals with familial hypercholesterolemia have reported that the efficacy of PSCK9 inhibitors such as evolocumab and alirocumab are affected by polymorphisms in apo B, low density lipoprotein receptor (LDL-R) and LDL-R adapter protein 1 genes (Hindi et al., 2021). Remarkably, a recent metanalysis provided evidence that the efficacy of PCSK9 inhibitors could be similar for different ethnicities (Sawant and Wang, 2023).
Figure 3: Mechanism of action of anti-proprotein convertase subtilisin kexin 9 (PCSK9) monoclonal antibodies. 1) PCSK9 binds to the low density lipoprotein receptor (LDLR) and directs it for degradation in lysosomes; 2) Anti-PCSK9 antibodies bind to PCSK9 and prevent it from attaching to LDLr; 3) Without PSK9 attached, LDLR can be recycled to the cell surface increasing the uptake of LDL particles from circulation.
The interaction between human PCSK9 and HDL particles remains a subject of ongoing debate, and research findings in this regard vary. Some recent studies have indeed indicated that PCSK9 binds to HDL in the bloodstream (Dafnis et al., 2022; Burnap et al., 2020), whereas another study did not find any association (Kosenko et al., 2013). An important question that has arisen in the context of PCSK9 inhibition is its potential effect on HDL function. Recent research has suggested that PCSK9 inhibitors may have a negative effect on HDL function, while other studies have found no such effect or even a beneficial effect on HDL function. In this context, an earlier study has proposed that PCSK9 hinders ABCA1-dependent cholesterol efflux from macrophages when apo A-I is present as the acceptor (Adorni et al., 2017a). Additionally, another study has demonstrated that PCSK9 impedes ABCA1-mediated cholesterol efflux from macrophages. This inhibition, attributed to the downregulation of the Abca1 gene and ABCA1 protein expression, implies that PCSK9 may have extrahepatic effects that can potentially impact key aspects of atherosclerosis development, such as the formation of foam cells. (Adorni et al., 2017b). In addition, a recent investigation has indicated that PCSK9 may have an impact on the functional properties of HDL. Specifically, it was found that PCSK9 reduces HDL’s ability to facilitate endothelial cell migration and hinder the formation of reactive oxygen species. Conversely, deactivating PCSK9 within HDL appears to enhance the atheroprotective functions of HDL (Dafnis et al., 2022). In contrast, Palumbo et al. (2022) evaluated a cohort of heterozygous familial hypercholesterolemic patients and observed that inhibition of PCSK9 has been shown to markedly enhance HDL cholesterol efflux, primarily through pathways involving ABCG1 and aqueous diffusion. Additionally, it reduces the serum’s ability to load macrophages with cholesterol, which is considered a proatherogenic marker and is associated with an elevated CV risk (Adorni et al., 2019).
About 90% of esterified cholesterol in plasma is synthesized by LCAT. Thus, LCAT plays a crucial role in HDL metabolism and RCT (Vitali and Cuchel, 2021). However, studies in humans and animals reported different results (Duan et al., 2022).
Recently, 2 different recombinant human LCAT enzymes (MEDI6012 and ACP-501) where shown to be safe in clinical studies including subjects with stable CVD (George et al., 2021; Shamburek et al., 2016). Hence, in a phase 2a double-blind study, 48 subjects with stable coronary heart disease were randomized on a statin to a single dose of MEDI6012 or placebo. Administration of recombinant LCAT resulted in dose-dependent increases in global and non-ABCA1 cell cholesterol efflux (George et al., 2021), suggesting that treatment with MEDI6012 may enhance RCT. In addition, in a phase I trial, infusion of ACP-501 was shown to increase HDL-C levels, cholesterol efflux and to favorably alter HDL metabolism, decreasing small and intermediate-sized HDL, and increasing large HDL particles (Shamburek et al., 2016).
More recent, findings suggest that, in addition to improving RCT, LCAT treatment may also improve other antiatherogenic properties of HDL, such as its ability to stimulate NO production from endothelial cells. HDL isolated from patients with myocardial infarction (MI) progressively lose the capacity to promote NO production by endothelial cells, and the reduction is related to decreased LCAT concentration. In vitro incubation of MI patients’ plasma with recombinant human LCAT restores HDL ability to promote endothelial NO production, possibly related to significant modification in HDL phospholipid classes (Ossoli et al., 2019).
It should be noted that both MEDI6012 and ACP-501 are administered subcutaneously, representing a noteworthy challenge in terms of patient adherence (Yang et al., 2022a). In addition, adverse effects of recombinant LCAT therapy seem to involve skin and subcutaneous issue disorders (Shamburek et al., 2016).
Statins are the most common drugs used for the treatment of dyslipidemia (Ward et al., 2019). Statins are selective inhibitors of β-hydroxy-β-methylglutaryl coenzyme A reductase (HMGCR), an enzyme involved in cholesterol synthesis (Ward et al., 2019). Fig. 4 shows statins mechanism of action. Side effects of statins include fibromyalgia, headache, dizziness and tiredness (Side Effects, 2022). Moreover, statins present harmful interactions with some immunosuppressors, protease inhibitors and antibiotics (Side Effects of Metformin, 2022). Most statins are orally administered once daily and peak plasma concentrations achieved in 1–3 h. It takes about 6 weeks before LDL-C levels are stabilized (Ward et al., 2019). Pharmacogenomics studies on statins have reported several genes that could influence both the response to these drugs and the apparition of adverse effects (Zineh, 2007; Canestaro et al., 2014). The effect of these genes would depend on the specific statin considered (Zineh, 2007). Similarly, polymorphisms in genes involved in lipid metabolism such as ABCA 1, LDL-R and HMGCR can influence the efficacy of statins (Hindi et al., 2021). Moreover, there is evidence of interracial differences in the response to statins (Lee et al., 2005). However, a later metanalysis reported that response to statins would be similar regardless of ethnicity (Sawant and Wang, 2023). On the other hand, statin effect on HDL functionality remains controversial (Muñoz-Hernandez et al., 2018; Lamon-Fava, 2013). In this regard, in a recent study, HDL proteome correlated with statin use, reporting a less antiatherogenic proteome in HDL3 particles from patients with type 1 diabetes mellitus (Toyoshima et al., 2023). On the other hand, in a study carried out in hypercholesterolemic patients both pravastatin and atorvastatin showed no effect on cholesterol efflux (Khera et al., 2011). In another study, atorvastatin failed to increase cholesterol efflux to total HDL, HDL2b and HDL3c in diabetic patients (Muñoz-Hernandez et al., 2018). Similarly, atorvastatin showed no effect in cholesterol efflux to apo B-depleted plasma in normolipidemic men (Ying et al., 2022). Furthermore, atorvastatin and simvastatin have been shown to decrease ABCA1 expression in macrophages and peripheral mononuclear cells (Qiu and Hill, 2008; Genvigir et al., 2010). Nevertheless, this effect could be abolished by loading the macrophages with cholesterol (Song et al., 2011). Moreover, statins would reduce the activity of CETP and PLTP, both involved in HDL metabolism and maturation (de Haan et al., 2008; Karalis et al., 2010). On the contrary, pitavastatin and, to a lesser extent, atorvastatin and simvastatin would increase ABCA1 expression in hepatocytes (Maejima et al., 2011). In fact, pitavastatin (but not atorvastatin) could increase ABCA1 half-life in hepatocytes (Kobayashi et al., 2011). Consistently, pitavastatin would increase HDL ability to promote cholesterol efflux and its antioxidant activity (Pirillo and Catapano, 2017; Miyamoto-Sasaki et al., 2013). In another study, atorvastatin alone or in combination with ezetimibe increased cholesterol efflux in a randomized clinical trial (Lee et al., 2017). Similarly, simvastatin with or without benzafibrate increased cholesterol efflux, but not HDL antioxidant and antiinflammatory activities in diabetic patients (Triolo et al., 2014). Moreover, rosuvastatin increased cholesterol efflux and PON 1 concentration in diabetic patients (Naresh et al., 2021). Consistently, in vitro studies show that statins could promote PON 1 activity (Marathe et al., 2019). Moreover, in a study carried out in vitro, rosuvastatin inhibited foam cell formation and stimulated ABCA1 and ABCG1 expression by promoting autophagy in macrophages (Zhang et al., 2021). Similarly, atorvastatin increased cholesterol efflux by promoting foam cell lipophagy (Zheng et al., 2021). Furthermore, pravastatin enhanced cholesterol efflux in chondrocytes (Wu et al., 2022). In summary, the effect of statin therapy on HDL functionality would depend on the statin employed and the target organ.
Figure 4: Mechanism of action of statins. Statins inhibit 3-hydroxy-3-methylglutaryl coenzyme A (HMG-CoA) reductase in hepatocytes. HMG-CoA reductase catalyzes the conversion of HMG-CoA into mevalonate, a key step for cholesterol synthesis, leading to reduced cholesterol availability. LDL, low density lipoprotein.
Another factor that could affect the response to statin therapy is the route of administration. Different strategies have been developed to improve the efficiency of drug-delivery to the target organ and prevent leakage in circulation. Indeed, in a recent study, copolymer modified atorvastatin-loaded discoidal rHDL strongly promoted macrophage differentiation into antiinflammatory M2 phenotype (Zhang et al., 2021). Similarly, LIPOSTAT, a simvastatin-loaded nanoliposome, was shown to enhance cholesterol efflux from foam cells (Rakshit et al., 2021). Furthermore, the combination of β-cyclodextrin and simvastatin-loaded rHDL in a sink/shuttle structure showed remarkable capacity to promote cholesterol efflux and decrease inflammation in macrophages (He et al., 2020). Importantly, the effect of statins on HDL functionality could extend beyond CVD therapy and be useful for the treatment of certain cancers and conditions such as osteoarthritis and glaucoma (Wu et al., 2022; Yang et al., 2022b; Yang et al., 2023).
Pioglitazone is an antidiabetic medication (Smith, 2001). It is administered orally once a day and peak plasma concentration is achieved 2 h after administration (Pioglitazone, 2017). Similar to statins, it takes several weeks before the desired effect can be achieved (Smith, 2001). Adverse effects of pioglitazone include headache, vision loss, pain or difficulty urinating, muscle pain, and weight gain among others (Pioglitazone, 2017). Pioglitazone selectively stimulates PPAR-α and PPAR-γ (Smith, 2001). Pharmacogenomic studies show that polymorphisms in PPAR-γ, Adiponectin, resistin, lipoprotein lipase (LPL), tumor necrosis factor-alpha and interleukin 6 (Il-6) genes could potentially influence the response to pioglitazone (Kawaguchi-Suzuki and Frye, 2013). The fact that pioglitazone regulates PPAR-α raises the possibility that this drug could affect HDL functionality, considering that the major role of PPAR-α deals with regulating HDL metabolism (Yoon, 2009). Indeed, there is evidence that pioglitazone could increase HDL functionality. In a study carried out in vitro, pioglitazone stimulated apo A-I secretion in HepG2 cells (Qin et al., 2007). These pioglitazone-induced apo A-I particles were capable of promoting cholesterol efflux from THP 1 macrophages (Qin et al., 2007). In another study pioglitazone increased ABCA1 expression in THP 1 macrophages (Liu et al., 2014). Furthermore, pioglitazone was capable of enhancing cholesterol efflux to HDL via both ABCA1 and ABCG1 transporters in vitro and ex vivo, employing sera from diabetic patients (Ozasa et al., 2011). This effect of pioglitazone would depend on PPAR-α activation (Ozasa et al., 2011). Furthermore, pioglitazone enhanced cholesterol efflux from macrophages by simulating PPAR-γ in mice (Ye et al., 2019). On the contrary, in another study, PPAR-γ stimulation by pioglitazone reduced ABCA1 expression in macrophages (Jiang and Li, 2017). In another study, pioglitazone stimulated cholesterol efflux and ABCA1 expression in gallbladder epithelial cells (Wang et al., 2015). In summary, the effect of pioglitazone on cholesterol efflux would depend on the type of cell and pathway involved. In addition to cholesterol efflux, pioglitazone could restore LCAT activity (Mirmiranpour et al., 2013).
Some studies have provided evidence that pioglitazone could also affect HDL antioxidant capacity. Both in humans and mouse models pioglitazone improved PON 1 activity (Mirmiranpour et al., 2013; Mahmoudi et al., 2019). In fact, pioglitazone was also proved to affect HDL resistance to oxidation (McEneny et al., 2013).
Metformin is usually employed as the first-line medication against type 2 diabetes. In addition, large clinical trials have shown that metformin can counteract diabetes-associated CV complications (He et al., 2019). Metformin is administered orally once or twice a day (He et al., 2019). Peak plasma concentrations are observed 3 h. and glucose lowering approximately 8 weeks after administration (He et al., 2019). Side effects of metformin include nausea, diarrhea, stomach ache and vitamin B 12 deficiency among others (NIH, 2022). In addition, metformin can interact with other antidiabetic medication such as insulin, plus with some diuretics and corticosteroids (NIH, 2022). Evidence suggests that the heritability of metformin response ranges between 20% and 34% (Florez, 2017). Nevertheless, the genetic markers identified thus far account for a small fraction of the variability observed in response to stains (Florez, 2017; Damanhouri et al., 2023). These findings suggest that many other genetic determinants of metformin action remain to be discovered and further pharmacogenomics studies are needed. The mechanism responsible for metformin-induced reduction of CV complications is still unknown. In this regard, different studies have suggested a positive effect of metformin on HDL functionality. In a study carried out in THP 1 human macrophages, metformin blunted foam cell formation induced by palmitic acid (Song et al., 2010). Moreover, in another study, metformin reduced foam cell formation, increased cholesterol efflux and upregulated both ABCA1 and ABCG1 in macrophages (Damanhouri et al., 2023). Metformin-mediated upregulation of ABCA1 and ABCG1 would depend on stimulation of fibroblast growth factor 21 (Luo et al., 2016). Similarly, metformin in combination with atorvastatin stimulated cholesterol efflux and both ABCA1 and ABCG1 expression in rabbits (Luo et al., 2017). In fact, metformin would be capable of restoring HDL ability to promote cholesterol efflux impaired by glycation (Matsuki et al., 2009). Furthermore, metformin could increase HDL-induced cholesterol efflux in vascular smooth muscle cells (Robichaud et al., 2022). This process would be mediated by autophagy induced by metformin (Robichaud et al., 2022). In another study, metformin inhibited foam cell formation by stimulating autophagy in macrophages from mice (Wu et al., 2021). Moreover, metformin could promote cholesterol efflux in chondrocytes (Xing et al., 2022). However, it should be noted that metformin did not induce changes in ABCA1 expression in a study carried out in diabetic patients (Fakhoury et al., 2022). Furthermore, metformin failed to promote cholesterol efflux in young individuals with type 1 diabetes (Gourgari et al., 2021).
Beyond RCT, evidence suggests that metformin could also affect HDL antioxidant activity. Metformin, alone or in combination with lycopene simulated PON 1 activity in obese mice (Motta et al., 2022). These results were replicated in diabetic rats for both metformin with or without lycopene (Figueiredo et al., 2020) and metformin with or without curcumin (Roxo et al., 2019). In fact, metformin-induced PON 1 activity resulted in a significant decrease in lipid peroxidation in diabetic rats (Wójcicka et al., 2016). Moreover, metformin could not only stimulate PON 1 activity, but also upregulate its expression (Camps et al., 2016). Furthermore, there is evidence that metformin could, via AMPK activation, improve HDL antioxidant and antiinflammatory activities in mice (Ma et al., 2017).
Ezetimibe is another LDL-C-lowering drug highly used in the clinical setting (Savarese et al., 2015). It interacts with Niemann–Pick C1-Like 1 (NPC1L1) protein to decrease intestinal absorption of LDL (Savarese et al., 2015; Phan et al., 2012). It is usually employed in combination with statins and capable of achieving a further 25% reduction of LDL-C compared to treatment with statins alone (Savarese et al., 2015). Ezetimibe is administered orally and peak concentration is achieved 4–12 h after administration (Drugbank, 2023a). LDL-C lowering is usually observed 2 weeks after the initiation of therapy (Side Effects of Ezetimibe, 2023). Its side effects include diarrhea, abdominal pain and tiredness (Side Effects of Ezetimibe, 2023). Genetic studies on the efficacy of ezetimibe suggest that it could be influenced by polymorphisms on genes associated with lipid metabolism such as NPC1L1, HMGCR and the transcription factor sterol regulatory binding protein 1 (Hindi et al., 2021). Interestingly, a recent metanalysis found that there are no major differences in the response to ezetimibe treatment among the different ethnicities (Sawant and Wang, 2023). Regarding its effect on HDL, Ezetimibe can produce modest increases in HDL-C (About Ezetimibe, 2023). In terms of HDL functionality, results are controversial. Ezetimibe was reported to increase PON 1 expression and activity in an animal model of non-alcoholic fatty liver disease (Hussein et al., 2012). On the contrary, ezetimibe reduced PON 1 activity in subjects who were receiving statin therapy (Tang et al., 2012). The fact that ezetimibe is usually administered with statins constitutes a confounding factor and further studies on ezetimibe effect over HDL are needed.
Fibrates are amphipathic derivatives of fibric acid (Grundy et al., 2005). Fibrates are employed to treat atherogenic dyslipemia characterized by high TG and low HDL-C levels (Grundy et al., 2005). Fibrates are administered orally and peak concentrations depend on the type of fibrate (Balendiran et al., 2007). Several weeks are needed before desired effects can be observed (Balendiran et al., 2007). Side effects include diarrhea, abdominal pain, dizziness, jaundice and headaches (Fibrates, 2022). Furthermore, fibrates can interact with blood thinners and immunosuppressors with harmful results (Fibrates, 2022). Fibrates stimulate peroxisome proliferator activated receptor alpha resulting in reduced fatty acids, TG and VLDL synthesis (Steiner, 2007). In parallel, production of Apo A-I and ABCA 1 is up-regulated (Steiner, 2007). Pharmacogenomic studies show that response to fibrates could be influenced by polymorphisms in PPAR-α, Apo E, Apo A-I, Apo B, Apo C-III, Apo AV, IL-6 and LPL genes (House and Motsinger-Reif, 2020). Furthermore, the efficacy of fibrates could also be affected by epigenetic modifications, specifically methylation levels, on the gene CEMIP2, which codifies a cell-surface transmembrane protein (House and Motsinger-Reif, 2020). Fibrates have been shown to be capable of increasing cholesterol efflux to HDL in animal models (Balendiran et al., 2007; Fournier et al., 2013). Nevertheless, studies carried out in humans have been contradictory (Gomaraschi et al., 2015). In a previous study fenofibrate increased ABCA 1 dependent cholesterol efflux to HDL in dyslipidemic patients (Franceschini et al., 2007). However, those results could not be replicated in a later study by the same group (Franceschini et al., 2013). In another study, fenofibrate also failed to increase cholesterol efflux to HDL (Maranghi et al., 2011). In contrast, bezafibrate increased cholesterol efflux in patients with type 2 diabetes (Triolo et al., 2014). Interestingly fenofibrate shows greater capacity to stimulate PON 1 activity than bezafibrate (Sahebkar et al., 2016). In summary, the effect of fibrates on HDL functionality would depend not only on the type of fibrate, but also on the specific function studied.
Lomitapide specifically inhibits microsomal triglyceride transport protein (MTP) (Ahn and Choi, 2015). MTP plays a crucial role in the assembly of VLDL particles (Ahn and Choi, 2015). As a result, lomitapide shows great efficiency at reducing both apo B and LDL-C levels (Ahn and Choi, 2015). Lomitapide is administered orally and peak concentrations are achieved in 6 h (Drugbank, 2023b). Lipid lowering is usually achieved after 6 weeks (Perry, 2013). Importantly, in addition to the usual diarrhea, dizziness and headaches, side effects of lomitapide include liver damage (Cuchel et al., 2007). Regarding HDL, lomitapide reduces HDL-C levels and evidence suggests it could also decrease ABCA 1 dependent cholesterol efflux, without changes in ABCG 1- and SRBI- mediated efflux (Yahya et al., 2016).
An innovative approach to the treatment of CVD involves targeting upstream processes, inhibiting the mRNA translation of proteins specifically implicated in lipid metabolism. Two categories of compounds have been primarily employed for this purpose: antisense oligonucleotides (ASO) and double-stranded short interfering RNA (siRNA) (Gareri et al., 2022). An important advantage of this approach is that it allows the targeting of several grams of a specific protein with a single RNA molecule, extending the intervals between doses (Gareri et al., 2022). Side effects depend on the specific therapy, but a main disadvantage of RNA-based drugs is that they need to be injected, potentially lowering patient adherence (Gareri et al., 2022). FDA-approved Mipomersen, an ASO that targets apo B, has shown remarkable capability to reduce apo-B, LDL-C, TG and non-HDL-C; however, it exerts no significant effect on HDL (Fogacci et al., 2019). Another FDA approved RNA-based drug, the previously mentioned Inclisiran, a siRNA targeted against PCSK9, has displayed the ability to increase HDL-C levels in addition to decreasing parameters associated with apo B-containing lipoproteins (Cupido and Kastelein, 2020). Similar results have been observed with Volanesorsen, an ASO directed against apo C-III (Kolovou et al., 2022). Nevertheless, the effect of these drugs on HDL functionality has not been tested. In this regard, evidence from animal models suggests that ASOs against angiopoietin-like protein 3, which inhibits endothelial lipase and is involved in HDL metabolism, increase RCT (Bell et al., 2021). Moreover, the same group reported previously that ASOs against CETP could also increase RCT (Bell et al., 2013). In conclusion, the focus of RNA-based therapy for CVD has been directed to molecules associated with apo B containing lipoproteins, developing RNA drugs for HDL-associated enzymes and proteins could be a fertile ground for new therapeutic developments.
Short synthetic peptides with sequences that mimic those found in natural apo A-I have been studied since the 1980’s for their potential to generate HDL-like nanoparticles and improve the function of endogenous HDL. These peptides are designed to replicate the behavior of apo A-I in promoting the formation and functions of endogenous HDL. Among the reported properties of apolipoprotein mimetic peptides are: 1) lipid-associating ability, 2) stimulation of HDL maturation and remodeling, 3) promotion of cholesterol efflux, and 4) antiinflammatory and antioxidant activities (Leman, 2015). However, it is important to note that most mimetics need to be administered intravenously, which constitutes a disadvantage when compared to drugs, such as statins, that can be administered orally. In addition, it should be noted that many of the studies with apo A-I mimetics have been carried out in mice, which do not naturally express CETP (Hogarth et al., 2003). The effect of CETP on apo A-I mimetics is unknown and makes extrapolation of results from animal models to humans difficult. Fig. 5 shows the effect of apo A-I mimetics on RCT.
Figure 5: Effect of apolipoprotein A-I mimetics on reverse cholesterol transport. Apolipoprotein (apo) A-I mimetics have the potential to increase cholesterol efflux from cells to high density lipoproteins (HDL) via ATP binding cassette subfamily (ABC) A1, ABCG1 and scavenger receptor class B type 1 (SRB1). Additionally, apo A-I mimetics can increase lecithin:cholesterol acyltransferase (LCAT) activity and HDL maturation. Mature HDL particles present increased lipid exchange with apo B containing lipoproteins via cholesteryl ester transfer protein (CETP), increasing cholesterol delivery to the liver. LDL, low density lipoprotein. LDLR, low density lipoprotein receptor.
The first apo A-I mimetics were based on the class A amphipathic helix structure that apo A-I residues 41 to 243 form (Smith, 2010). Two of the first mimetics developed were 18A and 37pA. 18A is the prototype 18-residue peptide, and 37pA is a dimer of two 18A peptides with a proline spacer (Anantharamaiah et al., 1985). Similar to apo A-I, these peptides can transform a phospholipid emulsion of dimyristoylphosphatidylcholine (DMPC), into disc-like rHDL particles (Anantharamaiah et al., 1985). The same group developed a series of more hydrophobic analogs with an increasing number of phenylalanine substitutions (referred to as 2F, 3F, 4F and 5F) (Datta et al., 2001). Several of these analogs exhibited increased lipid association activity and the 4F peptide demonstrated the strongest ability to absorb oxidized fatty acids and antiinflammatory activity (van Lenten et al., 2008). Similar to apo A-I, a considerable subset of these peptides has the potential to function as acceptors for cellular cholesterol through ABCA1. Nevertheless, it is noteworthy that when present in elevated concentrations, these peptides manifest detergent-like characteristics and possess the capability to extricate cholesterol from cells in an ABCA1-independent manner (Remaley et al., 2003). Remarkably, 5F peptide reduced aortic root atherosclerosis when injected to C57BL/6 mice fed a high cholesterol atherogenic diet (Garber et al., 2001).
Peptide 4F has been the most studied of apo A-I mimetics and has shown the most promising results to date. APP-018, an analog of 4F which can be administered orally, reduced aortic root lesion in apo E-deficient mice (Navab et al., 2002). Moreover, APP-018 treatment increased small preβ-HDL levels and HDL antiinflammatory activity (Navab et al., 2004). In one study, APP-018 and statins were capable of triggering the regression of lesions in the aortic root and the entire aorta in apo E deficient mice (Navab et al., 2005). Nevertheless, in another study, these results could not be replicated when APP-018 was administered without statins in apo E-deficient mice (Li et al., 2004). Furthermore, in two other animal studies, 4F presented potent antiinflammatory activity (van Lenten et al., 2008; Navab et al., 2012). In a phase I clinical trial, which included 50 patients with coronary artery disease, APP-018 increased HDL antiinflammatory activity when administered orally in high doses (Bloedon et al., 2008). However, in another study, in which 4F was administered by intravenous infusion or by subcutaneous injection, 4F failed to raise HDL antiinflammatory or antioxidant activities (Watson et al., 2011). Interestingly, in a recent study, 4F restored HDL antioxidant activity when added to a mixture that contained dysfunctional HDL isolated from diabetic patients (Feng et al., 2022). Furthermore, 4F decreased oxidation and increased NO production in mice fed a western diet (Ning et al., 2021). In fact, 4F could protect against oxidation and inflammation even after hypoxia exposure (Paul et al., 2021). Moreover, evidence suggests that 4F protects against endothelial dysfunction (Jiang et al., 2021). Similarly, 4F could improve left-ventricular hypertrophy, which results from coronary artery disease, in rabbits (Kraler et al., 2021). Furthermore, 4F could inhibit cardiac remodeling after MI (Hamid et al., 2020).
Another peptide, ATI-5261, a single amphipathic helix, was shown to be highly efficient promoting cholesterol efflux and reducing plaque lipid content in apo E deficient mice (Bielicki et al., 2010). 6F is another apo A-I mimetic with potent antioxidant and antiinflammatory effects. Its main advantage over other mimetics is that it does not require end blocking for its production, raising the possibility that it could be synthetized by living organisms and facilitating large scale production (Chattopadhyay et al., 2013; Navab et al., 2013). In fact, tomatoes have been genetically engineered to express 6F. When fed to mice, these tomatoes have produced impressive reductions in atherogenic lessons (Chattopadhyay et al., 2013). The reason for these findings would be that oral administration of apo A-I mimetics is more effective due to the intestine being the main site of action (Hogarth et al., 2003). The apo A-I mimetic peptide 37pA is highly capable of inducing cholesterol efflux; however, its high lipid affinity makes it cytotoxic (Ning et al., 2021). As a result, another peptide named 5A was designed by replacing 5 amino acids of 37pA with alanine residues making it less cytotoxic, but retaining its capacity to promote cholesterol efflux (Sethi et al., 2008). In fact, 5A can promote efflux via both ABCA1 and ABCG1 transporters (Amar et al., 2010). Moreover, 5A presents antiinflammatory and antioxidant activities (Tabet et al., 2010). Another apo A-I mimetic is etc-642, a complex of a 22-amino acid peptide that forms an amphipathic helix and phospholipids. etc-642 causes a shift in HDL subfractions towards the highly antiatherogenic preß-HDL and increases cholesterol efflux and antiinflammatory activity by blunting the expression of necrosis factor (NF)-kB and endothelial adhesion molecules in endothelial cells (di Bartolo et al., 2011a, 2011b; Iwata et al., 2011).
D’Souza et al. (2010) conducted a noteworthy investigation into the impact of 22 distinct bihelical apolipoprotein A-I mimetic peptides on cholesterol efflux, as well as their anti-inflammatory and antioxidant properties. Intriguingly, none of the examined compounds exhibited superiority across all antiatherogenic functions. These results indicate that apo A-I mimetic peptides more closely resembling apo A-I do not necessarily improve all various antiatherosclerotic functions. Moreover, combining several apo A-I mimetic peptides, each mimicking different structural aspects of apo A-I, may prove to be a valuable strategy to mimic the various antiatherosclerotic properties of apo A-I (Stoekenbroek et al., 2015).
It is important to note, that apo A-I mimetics are currently being studied for the treatment of other diseases apart from CVD. The disease models in which apolipoprotein mimetic peptides have shown benefit include cancer (Hogarth et al., 2003), colitis (Hogarth et al., 2003), asthma (Ghosh et al., 2022), sickle cell disease (Reddy et al., 2014), insulin resistance (Reddy et al., 2014), endotoxemia (Reddy et al., 2014), lung fibrosis (Wygrecka et al., 2023), HIV infection (Daskou et al., 2022), Parkinson’s disease (Jiang and Bai, 2022), and cognitive function and Alzheimer’s disease (Hogarth et al., 2003; Vanherle et al., 2022). Moreover, 4F was shown to inhibit in vitro replication of SARS-COV-2 (Kelesidis et al., 2021).
In summary, apo A-I mimetics are a promising tool for the treatment not only of CVD and related complications, but also of a number of other diseases. In fact, in recent years, researchers have produced mimetics for other apolipoproteins, such as apo E, apo C-III and apo J. Overall, there are currently 60 different peptides that have been approved for human use and over 150 peptides that are in various stages of clinical development (Wolska et al., 2021).
HDL is highly heterogeneous and composed of hundreds of lipids and proteins. rHDL provides tools that allow a better understanding of HDL composition and its roles in CVD. Infusion of exogenous HDL is known as HDL replacement therapy. rHDL is synthesized by the combination of apo A-I (or its mimetics) and phospholipids (Huang et al., 2020). One of the first and most studied rHDL particles was etc-216, composed of apo A-I Milano and phosphatidylcholine (POPC). Apo A-I Milano is a naturally occurring mutant of apo A-I that was shown to possess cardioprotective properties superior to wild type apo A-I (Franceschini et al., 1980). These particles increased cholesterol efflux and reduced atherosclerotic plaques in mice (Nicholls et al., 2011). Moreover, etc-216 showed higher antiinflammatory activity and plaque regression than wild type HDL in rabbits (Nissen et al., 2003; Ibanez et al., 2012). Unfortunately, clinical trials had to be stopped due to increases in neutrophile and decreases in lymphocyte count (Huang et al., 2020). As a result, another rHDL containing apo A-I Milano and POPC was designed and named MDCO-216. It was tested in patients with coronary artery disease and it showed none of the adverse effects of its predecessor whilst increasing cholesterol efflux (Reijers et al., 2017; Kempen et al., 2014). Moreover, MDCO-216 increased preß-HDL concentration and cholesterol efflux in healthy volunteers and patients with stable coronary artery disease (Kempen et al., 2016; Kallend et al., 2016). Nevertheless, MDCO-216 failed to trigger plaque regression in patients with acute coronary syndrome (Nicholls et al., 2018).
CER-001 is another rHDL made of recombinant human apo A-I, sphingomyelin (SPM), and dipalmitoylphosphatidyl glycerol. CER-001 increased cholesterol efflux, promoted plaque regression and decreased inflammation in animal studies (Tardy et al., 2014). Nevertheless, in a clinical trial, CER-001 failed to promote plaque regression 6 weeks after administration (Tardif et al., 2014). Similarly, CER-001 could not promote plaque regression in patients with acute coronary syndrome 10 weeks after administration in combination with statins (Nicholls et al., 2018). CSL-111 is a rHDL which contains wild type apo A-I and phospholipids. It was capable of reducing inflammation and improving post-ischemic heart function in mice (Richart et al., 2019; Bhushan et al., 2012, 2014). Nevertheless, CSL-111 could not achieve plaque regression and increased markers of liver damage in humans (Tardif et al., 2007). To avoid these adverse outcomes, a new formulation called CSL-112 was developed. It contains wild type apo A-I, POPC and sucrose serving as a stabilizing agent. CSL-112, when administered, demonstrated an augmentation in cholesterol efflux from macrophages (Diditchenko et al., 2013). Furthermore, it was shown to achieve plaque correction in two human clinical trials (Tardif et al., 2007; Waksman et al., 2010). A mayor phase III clinical trial is presently underway (Korjian et al., 2023). Fig. 6 shows the main rHDL preparations.
Figure 6: The five main reconstituted HDL preparations. Apo, apolipoprotein; HDL, high density lipoprotein.
The principal rHDL-based therapies enumerated earlier exhibit notable distinctions in their composition, dosing regimens, pharmacokinetic profiles, and pharmacodynamic properties. Numerous multifaceted factors could underlie the ineffectiveness of certain of these therapies. Initially, the study duration might have been insufficient to yield meaningful results. Additionally, the dosage employed might have been inadequate to achieve the desired therapeutic effect, or the frequency of administration may not have been sufficient. Furthermore, it is well-established that oxidative stress and inflammation have the capacity to modify the composition and functionality of HDL, potentially compromising its atheroprotective properties following administration (Huang et al., 2020). A recent meta-analysis which included studies carried out in both humans and animal models showed that HDL/apo A-I replacement therapy was not associated with decreases in atheroma volume in humans, but it did associate with changes in lesion area in mice (Abudukeremu et al., 2021). The authors conclude that further studies are necessary to explain the differences between animals and humans.
An important factor that could affect rHDL performance is the type of apo A-I employed. Indeed, in recent years, different formulations of rHDL which employ synthetic apo A-I mimetics instead of naturally occurring apo A-I have been developed. One of these preparations employs the aforementioned 18 A peptide, known for its antioxidant capacity (Hogarth et al., 2003). Other rHDL preparations have included the 5A peptide and have shown promising results in animal models by triggering plaque regression (Schwendeman et al., 2015; He et al., 2021). One preparation which has received much attention is etc-642, which includes the mimetic 22A, specifically engineered to activate LCAT. This preparation was shown to greatly increase HDL-C levels in dyslipidemic patients (van Capelleveen et al., 2014). In a recent study, authors tested the ability to promote cholesterol efflux and esterification of 3 of these preparations and found that the mimetics with the highest capacity to promote both steps of RCT in vitro could no replicate those results in vivo (Yuan et al., 2023). These findings underscore the significance of both pharmacodynamics and pharmacokinetics in determining the effectiveness of rHDL formulations. Similar to apo A-I mimetics, a main disadvantage of rHDL is the need to be administered intravenously, which could affect patient adherence (Huang et al., 2020).
In recent years, rHDL preparations have received great interest as delivery mechanisms for drugs and different biomolecules (Huang et al., 2020). Indeed, rHDL can efficiently deliver drugs to macrophages and atherosclerotic plaques. In mice, rHDL was capable of efficiently deliver simvastatin to atherosclerotic plaques, leading to a decrease in inflammation, plaque regression and an increase in the half-life of statins (Duivenvoorden et al., 2014). Moreover, rHDL particles have been developed for the delivery of antisense nucleotides and small interference RNA (Raut et al., 2018; Lacko et al., 2015). Fig. 7 shows the main properties of rHDL preparations.
Figure 7: Multiple properties of reconstituted high density lipoproteins. Reconstituted high density lipoproteins (rHDL) present several atheroprotective effects. Some rHDL present potent antioxidant activity leading to reduced radical oxygen species (ROS) production in cells and decreased oxidized LDL (oxLDL) formation. Furthermore, rHDL can inhibit the secretion of cytokines and adhesion molecules in different cell types. In addition, rHDL have remarkable capacity to promote cholesterol efflux and reverse cholesterol transport. Finally, rHDL represent an efficient vehicle for drug delivery to multiple target cells. PON 1, paraoxonase 1; apo, apolipoprotein; LPS, lipopolysaccharide; MCP-1, monocyte chemoattractant protein 1; VCAM-1, vascular cell adhesion molecule 1; ICAM-1, intercellular adhesion molecule 1; ABCA1, ATP binding cassette subfamily A1; ABCG1, ATP binding cassette subfamily G1; SRB1, scavenger receptor class B type 1.
Concluding Remarks and Future Perspectives
LDL-C levels have historically been the target for therapies aimed at reducing CVD risk. Nevertheless, despite the remarkable reductions in LDL-C levels achieved with drugs such as statins and PCSK9 inhibitors, significant residual risk persists. On the other hand, therapies aimed at increasing HDL-C have, for the most part, failed to achieve CVD reduction. When added together, these facts have made HDL functionality a therapeutic target of the utmost interest. Indeed, several drugs described in the present review have shown promising results in enhancing HDL functionality. Fig. 8 shows the main drugs capable of enhancing HDL functionality These drugs include molecules already approved for human use, such as statins and niacin, and, particularly, compounds currently undergoing development such as several apo A-I mimetics and rHDL preparations. Indeed, there are currently over 150 mimetic peptides at some stage of development, in addition to several rHDL preparations. These therapies show promising potential to specifically improve HDL functionality. Moreover, rHDL has the added benefit of notably improving drug delivery to the intended organ/tissue, enhancing therapeutic response. Nevertheless, pharmacogenomic data on these drugs is still scarce and further studies on the effect genetic variants have on their efficacy are needed. Moreover, studies analyzing ethnic differences in the response to these drugs are lacking and minorities are underrepresented. More genetic studies are necessary to establish a better therapeutic alternative both on a population and on an individual level. Nevertheless, evidence is strong and future therapeutic strategies should incorporate HDL functionality as a main target of interest, seeking a balance between these new parameters and traditional quantitative markers such as HDL-C, in order to achieve a better assessment of CVD risk and its management. Finally, the focus on HDL functionality could extend the benefits of these drugs beyond CVD management and into the treatment of several other illnesses, such as cancer, endocrine disorders, and autoimmune diseases.
Figure 8: Action of different therapies on HDL functionality. CETP, cholesteryl ester transfer protein; PCSK9, proprotein convertase subtilisin/kexin type 9; LCAT, lecithin:cholesteryl acyl transferase; apo, apolipoprotein; HDL, high density lipoprotein. *Controversy in different studies. Need of a deeper understanding of the mechanisms involved. **Depends on the statin employed.
Acknowledgement: None.
Funding Statement: The authors received no specific funding for this study.
Author Contributions: The authors confirm contribution to the paper as follows: Study conception and design: Leonardo Gómez Rosso, Belén Davico, Ezequiel Lozano Chiappe, Walter Tetzlaff, Laura Boero, Fernando Brites, and Maximiliano Martín; Data collection: Leonardo Gómez Rosso, Belén Davico, Ezequiel Lozano Chiappe, Walter Tetzlaff, Laura Boero, Fernando Brites, and Maximiliano Martín; Draft manuscript preparation: Leonardo Gómez Rosso, Belén Davico, Ezequiel Lozano Chiappe, Walter Tetzlaff, Laura Boero, Fernando Brites, and Maximiliano Martín; All authors reviewed the results and approved the final version of the manuscript..
Availability of Data and Materials: Data sharing not applicable to this article as no datasets were generated or analyzed during the current study.
Ethics Approval: Not applicable.
Conflicts of Interest: The authors declare that they have no conflicts of interest to report regarding the present study.
References
About Ezetimibe (2023). https://www.nhs.uk/medicines/ezetimibe/about-ezetimibe/ (accessed on 13/06/2023). [Google Scholar]
Abudukeremu A, Huang C, Li H, Sun R, Liu X et al. (2021). Efficacy and safety of high-density lipoprotein/apolipoprotein A1 replacement therapy in humans and mice with atherosclerosis: A systematic review and meta-analysis. Frontiers in Cardiovascular Medicine 8: 700233. [Google Scholar] [PubMed]
Adorni MP, Cipollari E, Favari E, Zanotti I, Zimetti F, Corsini A, Ricci C, Bernini F, Ferri N (2017a). Inhibitory effect of PCSK9 on Abca1 protein expression and cholesterol efflux in macrophages. Atherosclerosis 256: 1–6. [Google Scholar] [PubMed]
Adorni MP, Ferri N, Marchianò S, Trimarco V, Rozza F, Izzo R, Bernini F, Zimetti F (2017b). Effect of a novel nutraceutical combination on serum lipoprotein functional profile and circulating PCSK9. Therapeutics and Clinical Risk Management 13: 1555–1562. [Google Scholar] [PubMed]
Adorni MP, Zimetti F, Cangiano B, Vezzoli V, Bernini F et al. (2019). High-density lipoprotein function is reduced in patients affected by genetic or idiopathic hypogonadism. The Journal of Clinical Endocrinology & Metabolism 104: 3097–3107. [Google Scholar]
Ahn CH, Choi SH (2015). New drugs for treating dyslipidemia: Beyond statins. Diabetes & Metabolism Journal 39: 87–94. [Google Scholar]
Amar MJ, D’Souza W, Turner S, Demosky S, Sviridov D et al. (2010). 5A apolipoprotein mimetic peptide promotes cholesterol efflux and reduces atherosclerosis in mice. Journal of Pharmacology and Experimental Therapeutics 334: 634–641. [Google Scholar] [PubMed]
Anantharamaiah GM, Jones JL, Brouillette CG, Schmidt CF, Chung BH, Hughes TA, Bhown AS, Segrest JP (1985). Studies of synthetic peptide analogs of the amphipathic helix. Structure of complexes with dimyristoyl phosphatidylcholine. Journal of Biological Chemistry 260: 10248–10255. [Google Scholar] [PubMed]
Balendiran GK, Verma M, Perry E (2007). Chemistry of fibrates. Current Chemical Biology 1: 311–316. [Google Scholar] [PubMed]
Barter PJ, Brewer HBJr, Chapman MJ, Hennekens CH, Rader DJ, Tall AR (2003). Cholesteryl ester transfer protein: A novel target for raising HDL and inhibiting atherosclerosis. Arteriosclerosis, Thrombosis, and Vascular Biology 23: 160–167. [Google Scholar] [PubMed]
Barter PJ, Caulfield M, Eriksson M, Grundy SM, Kastelein JJ et al. (2007). Effects of torcetrapib in patients at high risk for coronary events. New England Journal of Medicine 357: 2109–2122. [Google Scholar] [PubMed]
Barter PJ, Nicholls S, Rye KA, Anantharamaiah GM, Navab M, Fogelman AM (2004). Antiinflammatory properties of HDL. Circulation Research 95: 764–772. [Google Scholar] [PubMed]
Bell TA3rd, Graham MJ, Lee RG, Mullick AE, Fu W, Norris D, Crooke RM (2013). Antisense oligonucleotide inhibition of cholesteryl ester transfer protein enhances RCT in hyperlipidemic, CETP transgenic, LDLr-/- mice. Journal of Lipid Research 54: 2647–2657. [Google Scholar] [PubMed]
Bell TA3rd, Liu M, Donner AJ, Lee RG, Mullick AE, Crooke RM (2021). Antisense oligonucleotide-mediated inhibition of angiopoietin-like protein 3 increases reverse cholesterol transport in mice. Journal of Lipid Research 62: 100101. [Google Scholar] [PubMed]
Bhushan S, Kondo K, Polhemus DJ, Otsuka H, Nicholson CK et al. (2014). Nitrite therapy improves left ventricular function during heart failure via restoration of nitric oxide-mediated cytoprotective signaling. Circulation Research 114: 1281–1291. [Google Scholar] [PubMed]
Bhushan S, Kondo K, Predmore BL, Zlatopolsky M, King AL, Pearce C, Huang H, Tao YX, Condit ME, Lefer DJ (2012). Selective β2-adrenoreceptor stimulation attenuates myocardial cell death and preserves cardiac function after ischemia-reperfusion injury. Arteriosclerosis, Thrombosis, and Vascular Biology 32: 1865–1874. [Google Scholar] [PubMed]
Bielicki JK, Zhang H, Cortez Y, Zheng Y, Narayanaswami V, Patel A, Johansson J, Azhar S (2010). A new HDL mimetic peptide that stimulates cellular cholesterol efflux with high efficiency greatly reduces atherosclerosis in mice. Journal of Lipid Research 51: 1496–1503. [Google Scholar] [PubMed]
Bloedon LT, Dunbar R, Duffy D, Pinell-Salles P, Norris R, DeGroot BJ, Movva R, Navab M, Fogelman AM, Rader DJ (2008). Safety, pharmacokinetics, and pharmacodynamics of oral apoA-I mimetic peptide D-4F in high-risk cardiovascular patients. Journal of Lipid Research 49: 1344–1352. [Google Scholar] [PubMed]
Bonizzi A, Piuri G, Corsi F, Cazzola R, Mazzucchelli S (2021). HDL dysfunctionality: Clinical relevance of quality rather than quantity. Biomedicines 9: 729. [Google Scholar] [PubMed]
Brites FD, Bonavita CD, de Geitere C, Cloës M, Delfly B, Yael MJ, Fruchart J, Wikinski RW, Castro GR (2000). Alterations in the main steps of reverse cholesterol transport in male patients with primary hypertriglyceridemia and low HDL-cholesterol levels. Atherosclerosis 152: 181–192. [Google Scholar] [PubMed]
Brites FD, Cavallero E, de Geitere C, Nicolaïew N, Jacotot B, Rosseneu M, Fruchart JC, Wikinski RL, Castro GR (1999). Abnormal capacity to induce cholesterol efflux and a new LpA-I pre-beta particle in type 2 diabetic patients. Clinica Chimica Acta 279: 1–14. [Google Scholar]
Brites F, Martin M, Guillas I, Kontush A (2017). Antioxidative activity of high-density lipoprotein (HDLInsights into potential clinical benefit. Biochimica et Biophysica Acta-Clinics 8: 66–77. [Google Scholar]
Brodeur MR, Rhainds D, Charpentier D, Mihalache-Avram T, Mecteau M et al. (2017). Dalcetrapib and anacetrapib differently impact HDL structure and function in rabbits and monkeys. Journal Lipid Research 58: 1282–1291. [Google Scholar]
Burnap SA, Joshi A, Tsimikas S, Fernández-Hernando C, Kiechl S, Berry SE, Hall W, Levkau B, Mayr M (2020). High-density lipoproteins are the main carriers of PCSK9 in the circulation. Journal of the American College of Cardiology 75: 1495–1497. [Google Scholar] [PubMed]
Calabresi L, Simonelli S, Gomaraschi M, Franceschini G (2012). Genetic lecithin: Cholesterol acyltransferase deficiency and cardiovascular disease. Atherosclerosis 222: 299–306. [Google Scholar] [PubMed]
Camont L, Chapman MJ, Kontush A (2011). Biological activities of HDL subpopulations and their relevance to cardiovascular disease. Trends in Molecular Medicine 17: 594–603. [Google Scholar] [PubMed]
Camps J, Hernandez-Aguilera A, Garcia-Heredia A, Cabre N, Luciano-Mateo F, Arenas M, Joven J (2016). Relationships between metformin, paraoxonase-1 and the chemokine (C-C Motif) ligand 2. Current Clinical Pharmacology 11: 250–258. [Google Scholar] [PubMed]
Canestaro WJ, Austin MA, Thummel KE (2014). Genetic factors affecting statin concentrations and subsequent myopathy: A HuGENet systematic review. Genetics in Medicine 16: 810–819. [Google Scholar] [PubMed]
Cardiovascular Diseases. https://www.who.int/health-topics/cardiovascular-diseases#tab=tab_1 (accessed on 02/03/2023). [Google Scholar]
Chapman MJ, Le Goff W, Guerin M, Kontush A (2010). Cholesteryl ester transfer protein: At the heart of the action of lipid-modulating therapy with statins, fibrates, niacin, and cholesteryl ester transfer protein inhibitors. European Heart Journal 31: 149–164. [Google Scholar] [PubMed]
Chattopadhyay A, Navab M, Hough G, Gao F, Meriwether D et al. (2013). A novel approach to oral apoA-I mimetic therapy. Journal of Lipid Research 54: 995–1010. [Google Scholar] [PubMed]
Cuchel M, Bloedon LT, Szapary PO, Kolansky DM, Wolfe ML et al. (2007). Inhibition of microsomal triglyceride transfer protein in familial hypercholesterolemia. New England Journal of Medicine 356: 148–156. [Google Scholar] [PubMed]
Cupido AJ, Kastelein JJP (2020). Inclisiran for the treatment of hypercholesterolaemia: Implications and unanswered questions from the ORION trials. Cardiovascular Research 116: e136–e139. [Google Scholar] [PubMed]
Dafnis I, Tsouka AN, Gkolfinopoulou C, Tellis CC, Chroni A, Tselepis AD (2022). PCSK9 is minimally associated with HDL but impairs the anti-atherosclerotic HDL effects on endothelial cell activation. Journal of Lipid Research 63: 100272. [Google Scholar] [PubMed]
Damanhouri ZA, Alkreathy HM, Alharbi FA, Abualhamail H, Ahmad MS (2023). A review of the impact of pharmacogenetics and metabolomics on the efficacy of metformin in type 2 diabetes. International Journal of Medical Sciences 20: 142–150. [Google Scholar] [PubMed]
Darabi M, Guillas-Baudouin I, Le Goff W, Chapman MJ, Kontush A (2016). Therapeutic applications of reconstituted HDL: When structure meets function. Pharmacology & Therapeutics 157: 28–42. [Google Scholar]
Daskou M, Mu W, Sharma M, Vasilopoulos H, Heymans R et al. (2022). ApoA-I mimetics reduce systemic and gut inflammation in chronic treated HIV. PLoS Pathogens 18: e1010160. [Google Scholar] [PubMed]
Datta G, Chaddha M, Hama S, Navab M, Fogelman AM et al. (2001). Effects of increasing hydrophobicity on the physical-chemical and biological properties of a class A amphipathic helical peptide. Journal of Lipid Research 42: 1096–1104. [Google Scholar] [PubMed]
Davidson WS, Shah AS, Sexmith H, Gordon SM (2022). The HDL proteome watch: Compilation of studies leads to new insights on HDL function. Biochimica et Biophysica Acta (BBA)—Molecular and Cell Biology of Lipids 1867: 159072. [Google Scholar] [PubMed]
de Haan W, van der Hoogt CC, Westerterp M, Hoekstra M, Dallinga-Thie GM, Princen HM, Romijn JA, Jukema JW, Havekes LM, Rensen PC (2008). Atorvastatin increases HDL cholesterol by reducing CETP expression in cholesterol-fed APOE*3-Leiden.CETP mice. Atherosclerosis 197: 57–63. [Google Scholar] [PubMed]
di Bartolo BA, Nicholls SJ, Bao S, Rye KA, Heather AK, Barter PJ, Bursill C (2011a). The apolipoprotein A-I mimetic peptide ETC-642 exhibits anti-inflammatory properties that are comparable to high density lipoproteins. Atherosclerosis 217: 395–400. [Google Scholar] [PubMed]
di Bartolo BA, Vanags LZ, Tan JT, Bao S, Rye KA, Barter PJ, Bursill CA (2011b). The apolipoprotein A-I mimetic peptide, ETC-642, reduces chronic vascular inflammation in the rabbit. Lipids in Health and Disease 10: 224. [Google Scholar] [PubMed]
Diditchenko S, Gille A, Pragst I, Stadler D, Waelchli M, Hamilton R, Leis A, Wright SD (2013). Novel formulation of a reconstituted high-density lipoprotein (CSL112) dramatically enhances ABCA1-dependent cholesterol efflux. Arteriosclerosis, Thrombosis, and Vascular Biology 33: 2202–2211. [Google Scholar] [PubMed]
Djadjo S, Bajaj T (2023). Niacin. In: 2022 StatPearls [Internet]. Treasure Island (FLStatPearls Publishing. [Google Scholar]
Dobiásová M, Frohlich JJ (1999). Advances in understanding of the role of lecithin cholesterol acyltransferase (LCAT) in cholesterol transport. Clinica Chimica Acta 286: 257–271. [Google Scholar]
Duan Y, Gong K, Xu S, Zhang F, Meng X, Han J (2022). Regulation of cholesterol homeostasis in health and diseases: From mechanisms to targeted therapeutics. Signal Transduction and Targeted Therapy 7: 265. [Google Scholar] [PubMed]
Drugbank (2023a). https://go.drugbank.com/drugs/DB00973 (accessed on 12/06/2023). [Google Scholar]
Duivenvoorden R, Tang J, Cormode DP, Mieszawska AJ, Izquierdo-Garcia D et al. (2014). A statin-loaded reconstituted high-density lipoprotein nanoparticle inhibits atherosclerotic plaque inflammation. Nature Communications 5: 3065. [Google Scholar] [PubMed]
Drugbank (2023b). https://go.drugbank.com/drugs/DB08827 (accessed on 12/06/2023). [Google Scholar]
Durairaj A, Sabates A, Nieves J, Moraes B, Baum S (2017). Proprotein convertase subtilisin/kexin type 9 (PCSK9) and its inhibitors: A review of physiology, biology, and clinical data. Current Treatment Options in Cardiovascular Medicine 19: 58. [Google Scholar] [PubMed]
D’Andrea E, Hey SP, Ramirez CL, Kesselheim AS (2019). Assessment of the role of niacin in managing cardiovascular disease outcomes: A systematic review and meta-analysis. JAMA Network Open 2: e192224. [Google Scholar] [PubMed]
D’Souza W, Stonik JA, Murphy A, Demosky SJ, Sethi AA, Moore XL, Chin-Dusting J, Remaley AT, Sviridov D (2010). Structure/function relationships of apolipoprotein a-I mimetic peptides: Implications for antiatherogenic activities of high-density lipoprotein. Circulation Research 107: 217–227. [Google Scholar] [PubMed]
Ebtehaj S, Gruppen EG, Bakker SJL, Dullaart RPF, Tietge UJF (2019). HDL (High-Density Lipoprotein) cholesterol efflux capacity is associated with incident cardiovascular disease in the general population. Arteriosclerosis, Thrombosis, and Vascular Biology 39: 1874–1883. [Google Scholar] [PubMed]
Emerging Risk Factors Collaboration, Di Angelantonio E, Sarwar N, Perry P, Kaptoge S, et al. (2009). Major lipids, apolipoproteins, and risk of vascular disease. JAMA 302: 1993–2000. [Google Scholar]
Fakhoury HMA, Elahi MA, Al Sarheed S, Al Dubayee M, Alshahrani A, Zhra M, Almassri A, Aljada A (2022). Gene expression profiling of peripheral blood mononuclear cells in type 2 diabetes: An exploratory study. Medicina 58: 1829. [Google Scholar] [PubMed]
Feng J, Wang Y, Li W, Zhao Y, Liu Y, Yao X, Liu S, Yu P, Li R (2022). High levels of oxidized fatty acids in HDL impair the antioxidant function of HDL in patients with diabetes. Frontiers in Endocrinology 13: 993193. [Google Scholar] [PubMed]
Fibrates (2022). https://medlineplus.gov/ency/patientinstructions/000789.htm (accessed on 12/06/2023). [Google Scholar]
Figueiredo ID, Lima TFO, Inácio MD, Costa MC, Assis RP, Brunetti IL, Baviera AM (2020). Lycopene improves the metformin effects on glycemic control and decreases biomarkers of glycoxidative stress in diabetic rats. Diabetes, Metabolic Syndrome and Obesity 13: 3117–3135. [Google Scholar] [PubMed]
Florez JC (2017). The pharmacogenetics of metformin. Diabetologia 60: 1648–1655. [Google Scholar] [PubMed]
Fogacci F, Ferri N, Toth PP, Ruscica M, Corsini A, Cicero AFG (2019). Efficacy and safety of mipomersen: A systematic review and meta-analysis of randomized clinical trials. Drugs 79: 751–766. [Google Scholar] [PubMed]
Fournier N, Tuloup-Minguez V, Pourci ML, Thérond P, Jullian JC, Wien F, Leroy M, Dallongeville J, Paul JL, Leroy A (2013). Fibrate treatment induced quantitative and qualitative HDL changes associated with an increase of S.R.-BI cholesterol efflux capacities in rabbits. Biochimie 95: 1278–1287. [Google Scholar] [PubMed]
Franceschini G, Calabresi L, Colombo C, Favari E, Bernini F, Sirtori CR (2007). Effects of fenofibrate and simvastatin on HDL-related biomarkers in low-HDL patients. Atherosclerosis 195: 385–391. [Google Scholar] [PubMed]
Franceschini G, Favari E, Calabresi L, Simonelli S, Bondioli A et al. (2013). Differential effects of fenofibrate and extended-release niacin on high-density lipoprotein particle size distribution and cholesterol efflux capacity in dyslipidemic patients. Journal of Clinical Lipidology 7: 414–422. [Google Scholar] [PubMed]
Franceschini G, Sirtori CR, Capurso2nd A, Weisgraber KH, Mahley RW (1980). A-I Milano apoprotein. Decreased high density lipoprotein cholesterol levels with significant lipoprotein modifications and without clinical atherosclerosis in an Italian family. Journal of Clinical Investigation 66: 892–900. [Google Scholar] [PubMed]
Furtado JD, Ruotolo G, Nicholls SJ, Dullea R, Carvajal-Gonzalez S, Sacks FM (2022). Pharmacological inhibition of CETP (Cholesteryl Ester Transfer Protein) increases HDL (High-Density Lipoprotein) that contains ApoC3 and other HDL subspecies associated with higher risk of coronary heart disease. Arteriosclerosis, Thrombosis, and Vascular Biology 42: 227–237. [Google Scholar] [PubMed]
Ganjali S, Ricciuti B, Pirro M, Butler AE, Atkin SL, Banach M, Sahebkar A (2019). High-density lipoprotein components and functionality in cancer: State-of-the-art. Trends in Endocrinology & Metabolism 30: 12–24. [Google Scholar]
Garber DW, Datta G, Chaddha M, Palgunachari MN, Hama SY, Navab M, Fogelman AM, Segrest JP, Anantharamaiah GM (2001). A new synthetic class A amphipathic peptide analogue protects mice from diet-induced atherosclerosis. Journal of Lipid Research 42: 545–552. [Google Scholar] [PubMed]
Gareri C, Polimeni A, Giordano S, Tammè L, Curcio A, Indolfi C (2022). Antisense oligonucleotides and small interfering RNA for the treatment of dyslipidemias. Journal of Clinical Medicine 11: 3884. [Google Scholar] [PubMed]
Genvigir FD, Rodrigues AC, Cerda A, Arazi SS, Willrich MA et al. (2010). Effects of lipid-lowering drugs on reverse cholesterol transport gene expressions in peripheral blood mononuclear and HepG2 cells. Pharmacogenomics 11: 1235–1246. [Google Scholar] [PubMed]
George RT, Abuhatzira L, Stoughton SM, Karathanasis SK, She D et al. (2021). MEDI6012: Recombinant human lecithin cholesterol acyltransferase, high-density lipoprotein, and low-density lipoprotein receptor-mediated reverse cholesterol transport. Journal of the American Heart Association 10: e014572. [Google Scholar] [PubMed]
Ghosh S, Rihan M, Ahmed S, Pande AH, Sharma SS (2022). Immunomodulatory potential of apolipoproteins and their mimetic peptides in asthma: Current perspective. Respiratory Medicine 204: 107007. [Google Scholar] [PubMed]
Goetze S, Frey K, Rohrer L, Radosavljevic S, Krützfeldt J, Landmesser U, Bueter M, Pedrioli PGA, von Eckardstein A, Wollscheid B (2021). Reproducible determination of high-density lipoprotein proteotypes. Journal of Proteome Research 20: 4974–4984. [Google Scholar] [PubMed]
Gomaraschi M, Adorni MP, Banach M, Bernini F, Franceschini G, Calabresi L (2015). Effects of established hypolipidemic drugs on HDL concentration, subclass distribution, and function. In: von Eckardstein A, Kardassis D, (eds). High Density Lipoproteins. Handbook of Experimental Pharmacology. vol. 224. Cham: Springer. [Google Scholar]
Gomez Rosso L, Lhomme M, Meroño T, Dellepiane A, Sorroche P et al. (2017). Poor glycemic control in type 2 diabetes enhances functional and compositional alterations of small, dense HDL3c. Biochimica et Biophysica Acta (BBA)—Molecular and Cell Biology of Lipids 1862: 188–195. [Google Scholar] [PubMed]
Gómez Rosso L, Benítez MB, Fornari MC, Berardi V, Lynch S, Schreier L, Wikinski R, Cuniberti L, Brites F (2008). Alterations in cell adhesion molecules and other biomarkers of cardiovascular disease in patients with metabolic syndrome. Atherosclerosis 199: 415–423. [Google Scholar]
Gómez Rosso L, Lhomme M, Meroño T, Sorroche P, Catoggio L et al. (2014). Altered lipidome and antioxidative activity of small, dense HDL in normolipidemic rheumatoid arthritis: Relevance of inflammation. Atherosclerosis 237: 652–660. [Google Scholar]
Gordon T, Castelli WP, Hjortland MC, Kannel WB, Dawber TR (1977). High density lipoprotein as a protective factor against coronary heart disease. The Framingham study. The American Journal of Medicine 62: 707–714. [Google Scholar] [PubMed]
Gourgari E, Nadeau KJ, Pyle L, Playford MP, Ma J, Mehta NN, Remaley AT, Gordon SM (2021). Effect of metformin on the high-density lipoprotein proteome in youth with type 1 diabetes. Endocrinology, Diabetes & Metabolism 4: e00261. [Google Scholar]
Grundy SM, Vega GL, Yuan Z, Battisti WP, Brady WE, Palmisano J (2005). Effectiveness and tolerability of simvastatin plus fenofibrate for combined hyperlipidemia (the SAFARI trial). American Journal of Cardiology 95: 462–468. [Google Scholar] [PubMed]
Gürgöze MT, Muller-Hansma AHG, Schreuder MM, Galema-Boers AMH, Boersma E, Roeters van Lennep JE (2019). Adverse events associated with PCSK9 inhibitors: A real-world experience. Clinical Pharmacology and Therapeutics 105: 496–504. [Google Scholar]
Habibe MN, Kellar JZ (2023). Niacin toxicity. In: StatPearls [Internet]. Treasure Island (FLStatPearls. https://www.ncbi.nlm.nih.gov/books/NBK559137/ (accessed on 12/06/2023). [Google Scholar]
Hafiane A, Genest J (2015). High density lipoproteins: Measurement techniques and potential biomarkers of cardiovascular risk. Biochimica et Biophysica Acta (BBA)—Clinical 3: 175–188. [Google Scholar]
Hamid T, Ismahil MA, Bansal SS, Patel B, Goel M, White CR, Anantharamaiah GM, Prabhu SD (2020). The apolipoprotein A-I mimetic L-4F attenuates monocyte activation and adverse cardiac remodeling after myocardial infarction. International Journal of Molecular Sciences 21: 3519. [Google Scholar] [PubMed]
He X, Chen X, Wang L, Wang W, Liang Q, Yi L, Wang Y, Gao Q (2019). Metformin ameliorates Ox-LDL-induced foam cell formation in raw264.7 cells by promoting ABCG-1 mediated cholesterol efflux. Life Sciences 216: 67–74. [Google Scholar] [PubMed]
He H, Hong K, Liu L, Schwendeman A (2021). Artificial high-density lipoprotein-mimicking nanotherapeutics for the treatment of cardiovascular diseases. Wiley Interdisciplinary Reviews: Nanomedicine and Nanobiotechnology 13: e1737. [Google Scholar] [PubMed]
He J, Yang Y, Zhou X, Zhang W, Liu J (2020). Shuttle/sink model composed of β-cyclodextrin and simvastatin-loaded discoidal reconstituted high-density lipoprotein for enhanced cholesterol efflux and drug uptake in macrophage/foam cells. Journal of Materials Chemistry B 8: 1496–1506. [Google Scholar] [PubMed]
Heinecke JW (2009). The HDL proteome: A marker—and perhaps mediator—of coronary artery disease. Journal of Lipid Research 50: S167–S171. [Google Scholar] [PubMed]
Hindi NN, Alenbawi J, Nemer G (2021). Pharmacogenomics variability of lipid-lowering therapies in familial hypercholesterolemia. Journal of Personalized Medicine 11: 877. [Google Scholar] [PubMed]
Hogarth CA, Roy A, Ebert DL (2003). Genomic evidence for the absence of a functional cholesteryl ester transfer protein gene in mice and rats. Comparative Biochemistry and Physiology. Part B, Biochemistry & Molecular Biology 135: 219–229. [Google Scholar]
House JS, Motsinger-Reif AA (2020). Fibrate pharmacogenomics: Expanding past the genome. Pharmacogenomics 21: 293–306. [Google Scholar] [PubMed]
HPS3/TIMI55–REVEAL Collaborative Group, Bowman L, Hopewell JC, Chen F, Wallendszus K et al. (2017). Effects of anacetrapib in patients with atherosclerotic vascular disease. New England Journal of Medicine 377: 1217–1227. [Google Scholar] [PubMed]
Huang J, Wang D, Huang LH, Huang H (2020). Roles of reconstituted high-density lipoprotein nanoparticles in cardiovascular disease: A new paradigm for drug discovery. International Journal of Molecular Sciences 21: 739. [Google Scholar] [PubMed]
Hung AM, Tsuchida Y, Nowak KL, Sarkar S, Chonchol M et al. (2019). IL-1 inhibition and function of the HDL-containing fraction of plasma in patients with stages 3 to 5 CKD. Clinical Journal of the American Society of Nephrology 14: 702–711. [Google Scholar] [PubMed]
Hussein O, Zidan J, Abu Jabal K, Shams I, Szvalb S, Grozovski M, Bersudsky I, Karry R, Aviram M (2012). Paraoxonase activity and expression is modulated by therapeutics in experimental rat nonalcoholic fatty liver disease. International Journal of Hepatology 2012: 265305. [Google Scholar] [PubMed]
Ibanez B, Giannarelli C, Cimmino G, Santos-Gallego CG, Alique M, Pinero A, Vilahur G, Fuster V, Badimon L, Badimon JJ (2012). Recombinant HDL(Milano) exerts greater anti-inflammatory and plaque stabilizing properties than HDL (wild-type). Atherosclerosis 220: 72–77. [Google Scholar] [PubMed]
Islam RM, Pourmousa M, Sviridov D, Gordon SM, Neufeld EB, Freeman LA, Perrin BSJr, Pastor RW, Remaley AT (2018). Structural properties of apolipoprotein A-I mimetic peptides that promote ABCA1-dependent cholesterol efflux. Scientific Reports 8: 2956. [Google Scholar] [PubMed]
Iwata A, Miura S, Zhang B, Imaizumi S, Uehara Y, Shiomi M, Saku K (2011). Antiatherogenic effects of newly developed apolipoprotein A-I mimetic peptide/phospholipid complexes against aortic plaque burden in Watanabe-heritable hyperlipidemic rabbits. Atherosclerosis 218: 300–307. [Google Scholar] [PubMed]
Jiang H, Bai X (2022). Apolipoprotein A-I mimetic peptides (ApoAI MP) improve oxidative stress and inflammatory responses in Parkinson’s disease mice. Frontiers in Pharmacology 13: 966232. [Google Scholar] [PubMed]
Jiang T, Du Q, Huang C, Xu W, Guo P, Li W, Xie X, Guo Y, Liu D, Lin D (2021). NMR-based metabolomic analysis on the protective effects of apolipoprotein A-I mimetic peptide against contrast media-induced endothelial dysfunction. Molecules 26: 5123. [Google Scholar] [PubMed]
Jiang M, Li X (2017). Activation of PPARγ does not contribute to macrophage ABCA1 expression and ABCA1-mediated cholesterol efflux to apoAI. Biochemical and Biophysical Research Communications 482: 849–856. [Google Scholar] [PubMed]
Kallend DG, Reijers JA, Bellibas SE, Bobillier A, Kempen H, Burggraaf J, Moerland M, Wijngaard PL (2016). A single infusion of MDCO-216 (ApoA-1 Milano/POPC) increases ABCA1-mediated cholesterol efflux and pre-beta 1 HDL in healthy volunteers and patients with stable coronary artery disease. European Heart Journal—Cardiovascular Pharmacotherapy 2: 23–29. [Google Scholar] [PubMed]
Kamanna VS, Ganji SH, Kashyap ML (2009). The mechanism and mitigation of niacin-induced flushing. International Journal of Clinical Practice 63: 1369–1377. [Google Scholar] [PubMed]
Karalis DG (2009). Intensive lowering of low-density lipoprotein cholesterol levels for primary prevention of coronary artery disease. Mayo Clinic Proceedings 84: 345–352. [Google Scholar] [PubMed]
Karalis IK, Bergheanu SC, Wolterbeek R, Dallinga-Thie GM, Hattori H, van Tol A, Liem AH, Wouter Jukema J (2010). Effect of increasing doses of Rosuvastatin and Atorvastatin on apolipoproteins, enzymes and lipid transfer proteins involved in lipoprotein metabolism and inflammatory parameters. Current Medical Research and Opinion 26: 2301–2313. [Google Scholar] [PubMed]
Kawaguchi-Suzuki M, Frye RF (2013). Current clinical evidence on pioglitazone pharmacogenomics. Frontiers in Pharmacology 4: 147. [Google Scholar] [PubMed]
Kelesidis T, Madhav S, Petcherski A, Cristelle H, O’Connor E, Hultgren NW, Ritou E, Williams DS, Shirihai OS, Reddy ST (2021). The ApoA-I mimetic peptide 4F attenuates in vitro replication of SARS-CoV-2, associated apoptosis, oxidative stress and inflammation in epithelial cells. Virulence 12: 2214–2227. [Google Scholar] [PubMed]
Kempen HJ, Asztalos BF, Moerland M, Jeyarajah E, Otvos J, Kallend DG, Bellibas SE, Wijngaard PL (2016). High-density lipoprotein subfractions and cholesterol efflux capacities after infusion of MDCO-216 (Apolipoprotein A-I Milano/Palmitoyl-Oleoyl-Phosphatidylcholine) in healthy volunteers and stable coronary artery disease patients. Arteriosclerosis, Thrombosis, and Vascular Biology 36: 736–742. [Google Scholar] [PubMed]
Kempen HJ, Schranz DB, Asztalos BF, Otvos J, Jeyarajah E, Drazul-Schrader D, Collins HL, Adelman SJ, Wijngaard PL (2014). Incubation of MDCO-216 (ApoA-IMilano/POPC) with human serum potentiates ABCA1-mediated cholesterol efflux capacity, generates new prebeta-1 HDL, and causes an increase in HDL size. Journal of Lipids 2014: 923903. [Google Scholar] [PubMed]
Khera AV, Cuchel M, de la Llera-Moya M, Rodrigues A, Burke MF et al. (2011). Cholesterol efflux capacity, high-density lipoprotein function, and atherosclerosis. New England Journal of Medicine 364: 127–135. [Google Scholar] [PubMed]
Kobayashi M, Gouda K, Chisaki I, Ochiai M, Itagaki S, Iseki K (2011). Regulation mechanism of ABCA1 expression by statins in hepatocytes. European Journal of Pharmacology 662: 9–14. [Google Scholar] [PubMed]
Kolovou G, Kolovou V, Katsiki N (2022). Volanesorsen: A new era in the treatment of severe hypertriglyceridemia. Journal of Clinical Medicine 11: 982. [Google Scholar] [PubMed]
Kontush A, Lhomme M, Chapman MJ (2013). Unraveling the complexities of the HDL lipidome. Journal of Lipid Research 54: 2950–2963. [Google Scholar] [PubMed]
Korjian S, Kazmi SHA, Chi G, Kalayci A, Lee JJ et al. (2023). Biological basis and proposed mechanism of action of CSL112 (Apolipoprotein A-I [Human]) for prevention of major adverse cardiovascular events in patients with myocardial infarction. European Heart Journal—Cardiovascular Pharmacotherapy 14: pvad014. [Google Scholar]
Kosenko T, Golder M, Leblond G, Weng W, Lagace TA (2013). Low density lipoprotein binds to proprotein convertase subtilisin/kexin type-9 (PCSK9) in human plasma and inhibits PCSK9-mediated low density lipoprotein receptor degradation. Journal of Biological Chemistry 288: 8279–8288. [Google Scholar] [PubMed]
Kosmas CE, Martinez I, Sourlas A, Bouza KV, Campos FN, Torres V, Montan PD, Guzman E (2018). High-density lipoprotein (HDL) functionality and its relevance to atherosclerotic cardiovascular disease. Drugs Context 7: 212525. [Google Scholar] [PubMed]
Kraler S, Wenzl FA, Akhmedov A, Blaser MC, Aikawa E, Lüscher TF (2021). ApoA-I mimetics improve aortic stenosis-associated left-ventricular diastolic dysfunction but fail to benefit rabbit models with normal aortic valves. International Journal of Cardiology 332: 159–161. [Google Scholar] [PubMed]
Lacko AG, Sabnis NA, Nagarajan B, McConathy WJ (2015). HDL as a drug and nucleic acid delivery vehicle. Frontiers in Pharmacology 6: 247. [Google Scholar] [PubMed]
Lamon-Fava S (2013). Statins and lipid metabolism: An update. Current Opinion in Lipidology 24: 221–226. [Google Scholar] [PubMed]
Lee CJ, Choi S, Cheon DH, Kim KY, Cheon EJ et al. (2017). Effect of two lipid-lowering strategies on high-density lipoprotein function and some HDL-related proteins: A randomized clinical trial. Lipids in Health and Disease 16: 49. [Google Scholar] [PubMed]
Lee E, Ryan S, Birmingham B, Zalikowski J, March R, Ambrose H, Moore R, Lee C, Chen Y, Schneck D (2005). Rosuvastatin pharmacokinetics and pharmacogenetics in white and Asian subjects residing in the same environment. Clinical Pharmacology & Therapeutics 78: 330–341. [Google Scholar]
Leman LJ (2015). The potential of apolipoprotein mimetic peptides in the treatment of atherosclerosis. Journal of Clinical Lipidology 10: 215–217. [Google Scholar]
Li X, Chyu KY, Faria Neto JR, Yano J, Nathwani N, Ferreira C, Dimayuga PC, Cercek B, Kaul S, Shah PK (2004). Differential effects of apolipoprotein A-I-mimetic peptide on evolving and established atherosclerosis in apolipoprotein E-null mice. Circulation 110: 1701–1705. [Google Scholar] [PubMed]
Lincoff AM, Nicholls SJ, Riesmeyer JS, Barter PJ, Brewer HB et al. (2017). Evacetrapib and cardiovascular outcomes in high-risk vascular disease. New England Journal of Medicine 376: 1933–1942. [Google Scholar] [PubMed]
Linton MF, Yancey PG, Davies SS, Jerome WG, Linton EF, Song WL, Doran AC, Vickers KC (2019). The role of lipids and lipoproteins in atherosclerosis. In: Feingold KR, Anawalt B, Boyce A, Chrousos G, de Herder WW, Dhatariya K, Dungan K, Hershman JM, Hofland J, Kalra S et al., (eds). Endotext [Internet]. South Dartmouth (MAMDText.com, Inc, 2000. [Google Scholar]
Liu HY, Cui HB, Chen XM, Chen XY, Wang SH et al. (2014). Imbalanced response of ATP-binding cassette transporter A1 and CD36 expression to increased oxidized low-density lipoprotein loading contributes to the development of THP-1 derived foam cells. Journal of Biochemistry 155: 35–42. [Google Scholar] [PubMed]
Lukasova M, Malaval C, Gille A, Kero J, Offermanns S (2011). Nicotinic acid inhibits progression of atherosclerosis in mice through its receptor GPR109A expressed by immune cells. Journal of Clinical Investigation 121: 1163–1173. [Google Scholar] [PubMed]
Luo F, Guo Y, Ruan G, Li X (2016). Metformin promotes cholesterol efflux in macrophages by up-regulating FGF21 expression: A novel anti-atherosclerotic mechanism. Lipids in Health and Disease 15: 109. [Google Scholar] [PubMed]
Luo F, Guo Y, Ruan GY, Long JK, Zheng XL, Xia Q, Zhao SP, Peng DQ, Fang ZF, Li XP (2017). Combined use of metformin and atorvastatin attenuates atherosclerosis in rabbits fed a high-cholesterol diet. Scientific Reports 7: 2169. [Google Scholar] [PubMed]
Ma A, Wang J, Yang L, An Y, Zhu H (2017). AMPK activation enhances the anti-atherogenic effects of high density lipoproteins in apoE-/- mice. Journal of Lipid Research 58: 1536–1547. [Google Scholar] [PubMed]
Madsen CM, Varbo A, Tybjærg-Hansen A, Frikke-Schmidt R, Nordestgaard BG (2018). U-shaped relationship of HDL and risk of infectious disease: Two prospective population-based cohort studies. European Heart Journal 39: 1181–1190. [Google Scholar] [PubMed]
Maejima T, Sugano T, Yamazaki H, Yoshinaka Y, Doi T, Tanabe S, Nishimaki-Mogami T (2011). Pitavastatin increases ABCA1 expression by dual mechanisms: SREBP2-driven transcriptional activation and PPARα-dependent protein stabilization but without activating LXR in rat hepatoma McARH7777 cells. Journal of Pharmacological Sciences 116: 107–115. [Google Scholar] [PubMed]
Mahmoudi A, Ghatreh Samani K, Amini SA, Heidarian E (2019). Effects of pioglitazone on the lipid profile, serum antioxidant capacity, and UCP1 gene expression in mouse brown adipose tissue. The Reports of Biochemistry & Molecular Biology 8: 15–20. [Google Scholar]
Mani P, Rohatgi A (2015). Niacin therapy, HDL cholesterol, and cardiovascular disease: Is the HDL hypothesis defunct? Current Atherosclerosis Reports 17: 43. [Google Scholar] [PubMed]
Maranghi M, Hiukka A, Badeau R, Sundvall J, Jauhiainen M, Taskinen MR (2011). Macrophage cholesterol efflux to plasma and HDL in subjects with low and high homocysteine levels: A FIELD substudy. Atherosclerosis 219: 259–265. [Google Scholar] [PubMed]
Marathe A, Ganaraja B, Ashwin Shenoy K, Ashok Prabhu K, Nandini M (2019). Effect of atorvastatin on serum para-oxonase-1 and C-reactive protein in wistar rats. Indian Journal of Clinical Biochemistry 34: 312–317. [Google Scholar] [PubMed]
Marsche G (2015). It’s time to reassess the high-density lipoprotein (HDL) hypothesis: CSL112, a novel promising reconstituted HDL formulation. Journal of the American Heart Association 4: e002371. [Google Scholar] [PubMed]
Martin M, Gaete L, Tetzlaff W, Ferraro F, Lozano Chiappe E et al. (2022). Vascular inflammation and impaired reverse cholesterol transport and lipid metabolism in obese children and adolescents. Nutrition, Metabolism and Cardiovascular Diseases 32: 258–268. [Google Scholar] [PubMed]
Masana L, Cabré A, Heras M, Amigó N, Correig X et al. (2015). Remarkable quantitative and qualitative differences in HDL after niacin or fenofibrate therapy in type 2 diabetic patients. Atherosclerosis 238: 213–219. [Google Scholar] [PubMed]
Matsuki K, Tamasawa N, Yamashita M, Tanabe J, Murakami H, Matsui J, Imaizumi T, Satoh K, Suda T (2009). Metformin restores impaired HDL-mediated cholesterol efflux due to glycation. Atherosclerosis 206: 434–438. [Google Scholar] [PubMed]
McEneny J, McPherson PA, McGinty A, Hull SS, McCance DR, Young IS (2013). Pioglitazone protects HDL(2&3) against oxidation in overweight and obese men. Annals of Clinical Biochemistry 50: 20–24. [Google Scholar] [PubMed]
Meroño T, Brites F, Dauteuille C, Lhomme M, Menafra M et al. (2015). Metabolic alterations, HFE gene mutations and atherogenic lipoprotein modifications in patients with primary iron overload. Clinical Science (London) 128: 609–618. [Google Scholar]
Meroño T, Dauteuille C, Tetzlaff W, Martín M, Botta E et al. (2017). Oxidative stress, HDL functionality and effects of intravenous iron administration in women with iron deficiency anemia. Clinical Nutrition 36: 552–558. [Google Scholar]
Meroño T, Gómez L, Sorroche P, Boero L, Arbelbide J, Brites F (2011). High risk of cardiovascular disease in iron overload patients. European Journal of Clinical Investigation 41: 479–486. [Google Scholar]
Meroño T, Sorroche P, Gómez Rosso LA, Casañas L, Boero LE, Arbelbide JA, Brites FD (2010). Proatherogenic disturbances in lipoprotein profile, associated enzymes and transfer proteins in women with iron deficiency anaemia. Clinical Biochemistry 43: 416–423. [Google Scholar]
Metzinger MP, Saldanha S, Gulati J, Patel KV, El-Ghazali A, Deodhar S, Joshi PH, Ayers C, Rohatgi A (2020). Effect of anacetrapib on cholesterol efflux capacity: A substudy of the DEFINE trial. Journal of the American Heart Association 9: e018136. [Google Scholar] [PubMed]
Miller GJ, Miller NE (1975). Plasma-high-density-lipoprotein concentration and development of ischaemic heart-disease. Lancet 1: 16–19. [Google Scholar] [PubMed]
Mineo C, Deguchi H, Griffin JH, Shaul PW (2006). Endothelial and antithrombotic actions of HDL. Circulation Research 98: 1352–1364. [Google Scholar] [PubMed]
Mirmiranpour H, Mousavizadeh M, Noshad S, Ghavami M, Ebadi M, Ghasemiesfe M, Nakhjavani M, Esteghamati A (2013). Comparative effects of pioglitazone and metformin on oxidative stress markers in newly diagnosed type 2 diabetes patients: A randomized clinical trial. Journal of Diabetes Complications 27: 501–507. [Google Scholar]
Miyamoto-Sasaki M, Yasuda T, Monguchi T, Nakajima H, Mori K, Toh R, Ishida T, Hirata K (2013). Pitavastatin increases HDL particles functionally preserved with cholesterol efflux capacity and antioxidative actions in dyslipidemic patients. Journal of Atherosclerosis and Thrombosis 20: 708–716. [Google Scholar] [PubMed]
Morton AM, Koch M, Mendivil CO, Furtado JD, Tjønneland A, Overvad K, Wang L, Jensen MK, Sacks FM (2018). Apolipoproteins E and CIII interact to regulate HDL metabolism and coronary heart disease risk. JCI Insight 3: e98045. [Google Scholar] [PubMed]
Motta BP, Pinheiro CG, Figueiredo ID, Cardoso FN, Oliveira JO, Machado RTA, da Silva PB, Chorilli M, Brunetti IL, Baviera AM (2022). Combined effects of lycopene and metformin on decreasing oxidative stress by triggering endogenous antioxidant defenses in diet-induced obese mice. Molecules 27: 8503. [Google Scholar] [PubMed]
Muñoz-Hernandez L, Ortiz-Bautista RJ, Brito-Córdova G, Lozano-Arvizu F, Saucedo S et al. (2018). Cholesterol efflux capacity of large, small and total HDL particles is unaltered by atorvastatin in patients with type 2 diabetes. Atherosclerosis 277: 72–79. [Google Scholar]
Naresh S, Bitla AR, Rao PVLNS, Sachan A, Amancharla YL (2021). Efficacy of oral rosuvastatin intervention on HDL and its associated proteins in men with type 2 diabetes mellitus. Endocrine 71: 76–86. [Google Scholar] [PubMed]
Navab M, Anantharamaiah GM, Hama S, Garber DW, Chaddha M, Hough G, Lallone R, Fogelman AM (2002). Oral administration of an Apo A-I mimetic Peptide synthesized from D-amino acids dramatically reduces atherosclerosis in mice independent of plasma cholesterol. Circulation 105: 290–292. [Google Scholar] [PubMed]
Navab M, Anantharamaiah GM, Hama S, Hough G, Reddy ST, Frank JS, Garber DW, Handattu S, Fogelman AM (2005). D-4F and statins synergize to render HDL antiinflammatory in mice and monkeys and cause lesion regression in old apolipoprotein E-null mice. Arteriosclerosis, Thrombosis, and Vascular Biology 25: 1426–1432. [Google Scholar] [PubMed]
Navab M, Anantharamaiah GM, Reddy ST, Hama S, Hough G et al. (2004). Oral D-4F causes formation of pre-beta high-density lipoprotein and improves high-density lipoprotein-mediated cholesterol efflux and reverse cholesterol transport from macrophages in apolipoprotein E-null mice. Circulation 109: 3215–3220. [Google Scholar] [PubMed]
Navab M, Hough G, Buga GM, Su F, Wagner AC et al. (2013). Transgenic 6F tomatoes act on the small intestine to prevent systemic inflammation and dyslipidemia caused by Western diet and intestinally derived lysophosphatidic acid. Journal of Lipid Research 54: 3403–3418. [Google Scholar] [PubMed]
Navab M, Reddy ST, Anantharamaiah GM, Hough G, Buga GM, Danciger J, Fogelman AM (2012). D-4F-mediated reduction in metabolites of arachidonic and linoleic acids in the small intestine is associated with decreased inflammation in low-density lipoprotein receptor-null mice. Journal of Lipid Research 53: 437–445. [Google Scholar] [PubMed]
Navab M, Reddy ST, Van Lenten BJ, Fogelman AM (2011). HDL and cardiovascular disease: Atherogenic and atheroprotective mechanisms. Nature Reviews Cardiology 8: 222–232. [Google Scholar] [PubMed]
Nelson AJ, Sniderman AD, Ditmarsch M, Dicklin MR, Nicholls SJ, Davidson MH, Kastelein JJP (2022). Cholesteryl ester transfer protein inhibition reduces major adverse cardiovascular events by lowering apolipoprotein B levels. International Journal of Molecular Sciences 23: 9417. [Google Scholar] [PubMed]
Niacin-Health Professional (2022). https://ods.od.nih.gov/factsheets/Niacin-HealthProfessional/ (accessed on 12/06/2023). [Google Scholar]
Nicholls SJ, Puri R, Anderson T, Ballantyne CM, Cho L et al. (2016). Effect of evolocumab on progression of coronary disease in statin-treated patients: The GLAGOV randomized clinical trial. Journal of the American Medical Association 316: 2373–2384. [Google Scholar] [PubMed]
Nicholls SJ, Puri R, Ballantyne CM, Jukema JW, Kastelein JJP et al. (2018). Effect of infusion of high-density lipoprotein mimetic containing recombinant apolipoprotein A-I milano on coronary disease in patients with an acute coronary syndrome in the MILANO-PILOT trial: A randomized clinical trial. Journal of the American Medical Association Cardiology 3: 806–814. [Google Scholar] [PubMed]
Nicholls SJ, Ray KK, Ballantyne CM, Beacham LA, Miller DL, Ruotolo G, Nissen SE, Riesmeyer JS, ACCENTUATE Investigators (2017). Comparative effects of cholesteryl ester transfer protein inhibition, statin or ezetimibe on lipid factors: The ACCENTUATE trial. Atherosclerosis 261: 12–18. [Google Scholar] [PubMed]
Nicholls SJ, Ruotolo G, Brewer HB, Kane JP, Wang MD, Krueger KA, Adelman SJ, Nissen SE, Rader DJ (2015). Cholesterol efflux capacity and pre-beta-1 HDL concentrations are increased in dyslipidemic patients treated with evacetrapib. Journal of the American College of Cardiology 66: 2201–2210. [Google Scholar] [PubMed]
Nicholls SJ, Uno K, Kataoka Y, Nissen SE (2011). ETC-216 for coronary artery disease. Expert Opinion on Biological Therapy 11: 387–394. [Google Scholar] [PubMed]
Ning DS, Ma J, Peng YM, Li Y, Chen YT et al. (2021). Apolipoprotein A-I mimetic peptide inhibits atherosclerosis by increasing tetrahydrobiopterin via regulation of GTP-cyclohydrolase 1 and reducing uncoupled endothelial nitric oxide synthase activity. Atherosclerosis 328: 83–91. [Google Scholar] [PubMed]
Nissen SE, Tsunoda T, Tuzcu EM, Schoenhagen P, Cooper CJ et al. (2003). Effect of recombinant ApoA-I milano on coronary atherosclerosis in patients with acute coronary syndromes: A randomized controlled trial. Journal of the American Medical Association 290: 2292–2300. [Google Scholar] [PubMed]
Ossoli A, Simonelli S, Varrenti M, Morici N, Oliva F et al. (2019). Recombinant LCAT (Lecithin: Cholesterol Acyltransferase) rescues defective HDL (High-Density Lipoprotein)-mediated endothelial protection in acute coronary syndrome. Arteriosclerosis, Thrombosis and Vascular Biology 39: 915–924. [Google Scholar] [PubMed]
Ozasa H, Ayaori M, Iizuka M, Terao Y, Uto-Kondo H et al. (2011). Pioglitazone enhances cholesterol efflux from macrophages by increasing ABCA1/ABCG1 expressions via PPARγ/LXRα pathway: Findings from in vitro and ex vivo studies. Atherosclerosis 219: 141–150. [Google Scholar] [PubMed]
Palumbo M, Giammanco A, Purrello F, Pavanello C, Mombelli G et al. (2022). Effects of PCSK9 inhibitors on HDL cholesterol efflux and serum cholesterol loading capacity in familial hypercholesterolemia subjects: A multi-lipid-center real-world evaluation. Frontiers in Molecular Biosciences 9: 925587. [Google Scholar] [PubMed]
Paul S, Gangwar A, Bhargava K, Ahmad Y (2021). D4F prophylaxis enables redox and energy homeostasis while preventing inflammation during hypoxia exposure. Biomedicine & Pharmacotherapy 133: 111083. [Google Scholar]
Perry CM (2013). Lomitapide: A review of its use in adults with homozygous familial hypercholesterolemia. American Journal of Cardiovascular Drugs 13: 285–296. [Google Scholar] [PubMed]
Phan BA, Dayspring TD, Toth PP (2012). Ezetimibe therapy: Mechanism of action and clinical update. Vascular Health Risk Management 8: 415–427. [Google Scholar] [PubMed]
Pierini FS, Botta E, Soriano ER, Martin M, Boero L et al. (2021). Effect of tocilizumab on LDL and HDL characteristics in patients with rheumatoid arthritis. An observational study. Rheumatology and Therapy 8: 803–815. [Google Scholar] [PubMed]
Pioglitazone (2017). https://medlineplus.gov/druginfo/meds/a699016.html (accessed on 12/06/2023). [Google Scholar]
Pirillo A, Catapano AL (2017). Pitavastatin and HDL: Effects on plasma levels and function(s). Atherosclerosis Supplements 27: e1–e9. [Google Scholar] [PubMed]
Pownall HJ, Gotto AMJr (2019). Lipids and cardiovascular disease: Putting it all together. Methodist Debakey Cardiovascular Journal 15: 5–8. [Google Scholar] [PubMed]
Puri R, Nissen SE, Somaratne R, Cho L, Kastelein JJ et al. (2016). Impact of PCSK9 inhibition on coronary atheroma progression: Rationale and design of global assessment of plaque regression with a PCSK9 antibody as measured by intravascular ultrasound (GLAGOV). American Heart Journal 176: 83–92. [Google Scholar] [PubMed]
Qin S, Liu T, Kamanna VS, Kashyap ML (2007). Pioglitazone stimulates apolipoprotein A-I production without affecting HDL removal in HepG2 cells: Involvement of PPAR-alpha. Arteriosclerosis, Thrombosis and Vascular Biology 27: 2428–2434. [Google Scholar] [PubMed]
Qiu G, Hill JS (2008). Atorvastatin inhibits ABCA1 expression and cholesterol efflux in THP-1 macrophages by an LXR-dependent pathway. Journal of Cardiovascular Pharmacology 51: 388–395. [Google Scholar] [PubMed]
Rakshit M, Darwitan A, Muktabar A, Das P, Nguyen LTH et al. (2021). Anti-inflammatory potential of simvastatin loaded nanoliposomes in 2D and 3D foam cell models. Nanomedicine 37: 102434. [Google Scholar] [PubMed]
Raut S, Dasseux JL, Sabnis NA, Mooberry L, Lacko A (2018). Lipoproteins for therapeutic delivery: Recent advances and future opportunities. Therapeutic Delivery 9: 257–268. [Google Scholar] [PubMed]
Ray KK, Troquay RPT, Visseren FLJ, Leiter LA, Scott Wright R et al. (2023). Long-term efficacy and safety of inclisiran in patients with high cardiovascular risk and elevated LDL cholesterol (ORION-3Results from the 4-year open-label extension of the ORION-1 trial. The Lancet Diabetes & Endocrinology 11: 109–119. [Google Scholar]
Reddy ST, Navab M, Anantharamaiah GM, Fogelman AM (2014). Apolipoprotein A-I mimetics. Current Opinion in Lipidology 25: 304–308. [Google Scholar] [PubMed]
Reijers JAA, Kallend DG, Malone KE, Jukema JW, Wijngaard PLJ, Burggraaf J, Moerland M (2017). MDCO-216 does not induce adverse immunostimulation, in contrast to its predecessor ETC-216. Cardiovascular Drugs and Therapy 31: 381–389. [Google Scholar] [PubMed]
Remaley AT, Thomas F, Stonik JA, Demosky SJ, Bark SE et al. (2003). Synthetic amphipathic helical peptides promote lipid efflux from cells by an ABCA1-dependent and an ABCA1-independent pathway. Journal of Lipid Research 44: 828–836. [Google Scholar] [PubMed]
Rena G, Hardie DG, Pearson ER (2017). The mechanisms of action of metformin. Diabetologia 60: 1577–1585. [Google Scholar] [PubMed]
Richart A, Reddy M, Natoli A, Heywood S, Khalaji M, Lancaster G, Diditchenko S, Navdaev A, Kingwell B (2019). ApoA-I nanoparticles (CSL-111) directly modulates inflammatory cells after myocardial infarction in mice. Arteriosclerosis, Thrombosis and Vascular Biology 39: A329. [Google Scholar]
Robichaud S, Rasheed A, Pietrangelo A, Doyoung Kim A, Boucher DM et al. (2022). Autophagy is differentially regulated in leukocyte and nonleukocyte foam cells during atherosclerosis. Circulation Research 130: 831–847. [Google Scholar] [PubMed]
Rohatgi A, Khera A, Berry JD, Givens EG, Ayers CR et al. (2014). HDL cholesterol efflux capacity and incident cardiovascular events. New England Journal of Medicine 371: 2383–2393. [Google Scholar] [PubMed]
Ronsein GE, Heinecke JW (2017). Time to ditch HDL-C as a measure of HDL function? Current Opinion in Lipidology 28: 414–418. [Google Scholar] [PubMed]
Ronsein GE, Vaisar T, Davidson WS, Bornfeldt KE, Probstfield JL, O’Brien KD, Zhao XQ, Heinecke JW (2021). Niacin increases atherogenic proteins in high-density lipoprotein of statin-treated subjects. Arteriosclerosis, Thrombosis and Vascular Biology 41: 2330–2341. [Google Scholar] [PubMed]
Roxo DF, Arcaro CA, Gutierres VO, Costa MC, Oliveira JO, Lima TFO, Assis RP, Brunetti IL, Baviera AM (2019). Curcumin combined with metformin decreases glycemia and dyslipidemia, and increases paraoxonase activity in diabetic rats. Diabetology & Metabolic Syndrome 11: 33. [Google Scholar]
Sacks FM, Jensen MK (2018). From high-density lipoprotein cholesterol to measurements of function: Prospects for the development of tests for high-density lipoprotein functionality in cardiovascular disease. Arteriosclerosis, Thrombosis and Vascular Biology 38: 487–499. [Google Scholar] [PubMed]
Sahebkar A, Hernández-Aguilera A, Abelló D, Sancho E, Camps J, Joven J (2016). Systematic review and meta-analysis deciphering the impact of fibrates on paraoxonase-1 status. Metabolism 65: 609–622. [Google Scholar] [PubMed]
Savarese G, de Ferrari GM, Rosano GM, Perrone-Filardi P (2015). Safety and efficacy of ezetimibe: A meta-analysis. International Journal of Cardiology 201: 247–252. [Google Scholar] [PubMed]
Savel J, Lafitte M, Pucheu Y, Pradeau V, Tabarin A, Couffinhal T (2012). Very low levels of HDL cholesterol and atherosclerosis, a variable relationship—A review of LCAT deficiency. Vascular Health Risk Management 8: 357–361. [Google Scholar] [PubMed]
Sawant S, Wang N (2023). Underrepresentation of ethnic and regional minorities in lipid-lowering randomized clinical trials: A systematic review and meta-analysis. European Journal of Preventive Cardiology 7: zwad030. [Google Scholar]
Schwartz GG, Olsson AG, Abt M, Ballantyne CM, Barter PJ et al. (2012). Effects of dalcetrapib in patients with a recent acute coronary syndrome. New England Journal of Medicine 367: 2089–2099. [Google Scholar] [PubMed]
Schwendeman A, Sviridov DO, Yuan W, Guo Y, Morin EE et al. (2015). The effect of phospholipid composition of reconstituted HDL on its cholesterol efflux and anti-inflammatory properties. Journal of Lipid Research 56: 1727–1737. [Google Scholar] [PubMed]
Sethi AA, Stonik JA, Thomas F, Demosky SJ, Amar M et al. (2008). Asymmetry in the lipid affinity of bihelical amphipathic peptides. A structural determinant for the specificity of ABCA1-dependent cholesterol efflux by peptides. Journal of Biological Chemistry 283: 32273–32282. [Google Scholar] [PubMed]
Shamburek RD, Bakker-Arkema R, Shamburek AM, Freeman LA, Amar MJ et al. (2016). Safety and tolerability of ACP-501, a recombinant human lecithin: Cholesterol acyltransferase, in a phase 1 single-dose escalation study. Circulation Research 118: 73–82. [Google Scholar] [PubMed]
Side Effects (2022). https://www.nhs.uk/conditions/statins/side-effects/ (accessed on 12/06/2023). [Google Scholar]
Side Effects of Ezetimibe (2023). https://www.nhs.uk/medicines/ezetimibe/side-effects-of-ezetimibe/ (accessed on 12/06/2023). [Google Scholar]
Side Effects of Metformin (2022). https://www.nhs.uk/medicines/metformin/side-effects-of-metformin/ (accessed on 13/06/2023). [Google Scholar]
Smith U (2001). Pioglitazone: Mechanism of action. International Journal of Clinical Practice Supplements 121: 13–18. [Google Scholar]
Smith JD (2010). Apolipoprotein A-I and its mimetics for the treatment of atherosclerosis. Current Opinion in Investigational Drugs 11: 989–996. [Google Scholar] [PubMed]
Song G, Liu J, Zhao Z, Yu Y, Tian H, Yao S, Li G, Qin S (2011). Simvastatin reduces atherogenesis and promotes the expression of hepatic genes associated with reverse cholesterol transport in apoE-knockout mice fed high-fat diet. Lipids in Health and Disease 10: 8. [Google Scholar] [PubMed]
Song J, Ren P, Zhang L, Wang XL, Chen L, Shen YH (2010). Metformin reduces lipid accumulation in macrophages by inhibiting FOXO1-mediated transcription of fatty acid-binding protein 4. Biochemical and Biophysical Research Communications 393: 89–94. [Google Scholar] [PubMed]
Steiner G (2007). Atherosclerosis in type 2 diabetes: A role for fibrate therapy? Diabetes & Vascular Disease Research 4: 368–374. [Google Scholar]
Stoekenbroek RM, Stroes ES, Hovingh GK (2015). ApoA-I mimetics. In: Handbook of Experimental Pharmacology, vol. 224, pp. 631–648. Springer. http://www.springer.com/series/164 [Google Scholar]
Suchowerska AK, Stokman G, Palmer JT, Coghlan PA, Pieterman EJ et al. (2022). A novel, orally bioavailable, small-molecule inhibitor of PCSK9 with significant cholesterol-lowering properties in vivo. Journal of Lipid Research 63: 100293. [Google Scholar] [PubMed]
Sugano M, Tsuchida K, Makino N (2000). High-density lipoproteins protect endothelial cells from tumor necrosis factor-alpha-induced apoptosis. Biochemical and Biophysical Research Communications 272: 872–876. [Google Scholar] [PubMed]
Sánchez-Pérez H, Quevedo-Abeledo JC, de Armas-Rillo L, Rua-Figueroa Í., Tejera-Segura B et al. (2020). Impaired HDL cholesterol efflux capacity in systemic lupus erythematosus patients is related to subclinical carotid atherosclerosis. Rheumatology 59: 2847–2856. [Google Scholar]
Tabet F, Remaley AT, Segaliny AI, Millet J, Yan L, Nakhla S, Barter PJ, Rye KA, Lambert G (2010). The 5A apolipoprotein A-I mimetic peptide displays antiinflammatory and antioxidant properties in vivo and in vitro. Arteriosclerosis, Thrombosis and Vascular Biology 30: 246–252. [Google Scholar] [PubMed]
Taheri H, Filion KB, Windle SB, Reynier P, Eisenberg MJ (2020). Cholesteryl ester transfer protein inhibitors and cardiovascular outcomes: A systematic review and meta-analysis of randomized controlled trials. Cardiology 145: 236–250. [Google Scholar] [PubMed]
Tall AR, Rader DJ (2017). Trials and tribulations of CETP inhibitors. Circulation Research 122: 106–112. [Google Scholar] [PubMed]
Tang W, Villines T, Hazen S, Stanek E, Hulten E, Taylor A (2012). Effect of niacin and ezetimibe on serum paraoxonase and arylesterase activities of hdl cholesterol. Journal of the American College of Cardiology 59: E1497. [Google Scholar]
Tardif JC, Grégoire J, L’Allier PL, Ibrahim R, Lespérance J et al. (2007). Effects of reconstituted high-density lipoprotein infusions on coronary atherosclerosis: A randomized controlled trial. Journal of the American Medical Association 297: 1675–1682. [Google Scholar] [PubMed]
Tardif JC, Ballantyne CM, Barter P, Dasseux JL, Fayad ZA et al. (2014). Effects of the high-density lipoprotein mimetic agent CER-001 on coronary atherosclerosis in patients with acute coronary syndromes: A randomized trial. European Heart Journal 35: 3277–3286. [Google Scholar] [PubMed]
Tardif JC, Pfeffer MA, Kouz S, Koenig W, Maggioni AP et al. (2022). Pharmacogenetics-guided dalcetrapib therapy after an acute coronary syndrome: The dal-GenE trial. European Heart Journal 43: 3947–3956. [Google Scholar] [PubMed]
Tardif JC, Rhainds D, Rhéaume E, Dubé MP (2017). CETP: Pharmacogenomics-based response to the CETP inhibitor dalcetrapib. Arteriosclerosis, Thrombosis and Vascular Biology 37: 396–400. [Google Scholar] [PubMed]
Tardif JC, Rhéaume E, Lemieux Perreault LP, Grégoire JC, Feroz Zada Y et al. (2015). Pharmacogenomic determinants of the cardiovascular effects of dalcetrapib. Circulation: Cardiovascular Genetics 8: 372–382. [Google Scholar] [PubMed]
Tardy C, Goffinet M, Boubekeur N, Ackermann R, Sy G et al. (2014). CER-001, a HDL-mimetic, stimulates the reverse lipid transport and atherosclerosis regression in high cholesterol diet-fed LDL-receptor deficient mice. Atherosclerosis 232: 110–118. [Google Scholar] [PubMed]
Timón-Zapata J, Laserna-Mendieta EJ, Pineda-Tenor D, Agudo-Macazaga M, Narros-Cecilia C, Rocha-Bogas MJ, Ruiz-Martín G, Gómez-Serranillos M (2011). Extreme concentrations of high density lipoprotein cholesterol affect the calculation of low density lipoprotein cholesterol in the Friedewald formula and other proposed formulas. Clinical Biochemistry 44: 1451–1456. [Google Scholar]
Toth PP (2003). Reverse cholesterol transport: High-density lipoprotein’s magnificent mile. Current Atherosclerosis Reports 5: 386–393. [Google Scholar] [PubMed]
Toyoshima MTK, Santana MFM, Silva ARM, Mello GB, Santos-Bezerra DP et al. (2023). Proteomics of high-density lipoprotein subfractions and subclinical atherosclerosis in type 1 diabetes mellitus: A case-control study. Diabetology & Metabolic Syndrome 15: 42. [Google Scholar]
Triolo M, Annema W, de Boer JF, Tietge UJ, Dullaart RP (2014). Simvastatin and bezafibrate increase cholesterol efflux in men with type 2 diabetes. European Journal of Clinical Investigation 44: 240–248. [Google Scholar] [PubMed]
Tsouka AN, Tellis CC, Tselepis AD (2018). Pharmacology of PCSK9 inhibitors: Current status and future perspectives. Current Pharmaceutical Design 24: 3622–3633. [Google Scholar] [PubMed]
Tuteja S, Qu L, Vujkovic M, Dunbar RL, Chen J, DerOhannessian S, Rader DJ (2018). Genetic variants associated with plasma lipids are associated with the lipid response to niacin. Journal of the American Heart Association 7: e03488. [Google Scholar] [PubMed]
van Capelleveen JC, Brewer HB, Kastelein JJ, Hovingh GK (2014). Novel therapies focused on the high-density lipoprotein particle. Circulation Research 114: 193–204. [Google Scholar] [PubMed]
van der Boom T, Jia C, Lefrandt JD, Connelly MA, Links TP, Tietge UJF, Dullaart RPF (2020). HDL cholesterol efflux capacity is impaired in severe short-term hypothyroidism despite increased HDL cholesterol. Journal of Clinical Endocrinology and Metabolism 105: e3355–e3362. [Google Scholar] [PubMed]
van Lenten BJ, Wagner AC, Jung CL, Ruchala P, Waring AJ et al. (2008). Anti-inflammatory apoA-I-mimetic peptides bind oxidized lipids with much higher affinity than human apoA-I. Journal of Lipid Research 49: 2302–2311. [Google Scholar] [PubMed]
Vanherle S, Jorissen W, Dierckx T, Loix M, Grajchen E et al. (2022). The ApoA-I mimetic peptide 5A enhances remyelination by promoting clearance and degradation of myelin debris. Cell Reports 41: 111591. [Google Scholar] [PubMed]
Vitali C, Cuchel M (2021). Controversial role of lecithin: Cholesterol acyltransferase in the development of atherosclerosis: New insights from an LCAT activator. Arteriosclerosis, Thrombosis and Vascular Biology 41: 377–379. [Google Scholar] [PubMed]
von Eckardstein A (2022). High density lipoproteins: Is there a comeback as a therapeutic target? In: von Eckardstein A, Binder CJ (edsPrevention and Treatment of Atherosclerosis. Handbook of Experimental Pharmacology, vol. 270, pp. 157–200. Springer, Cham. [Google Scholar]
Waksman R, Torguson R, Kent KM, Pichard AD, Suddath WO et al. (2010). A first-in-man, randomized, placebo-controlled study to evaluate the safety and feasibility of autologous delipidated high-density lipoprotein plasma infusions in patients with acute coronary syndrome. Journal of the American College of Cardiology 55: 2727–2735. [Google Scholar] [PubMed]
Walldius G, Jungner I (2007). Apolipoprotein A-I versus HDL cholesterol in the prediction of risk for myocardial infarction and stroke. Current Opinion in Cardiology 22: 359–367. [Google Scholar] [PubMed]
Wang JM, Wang D, Tan YY, Zhao G, Ji ZL (2015). Pioglitazone reduces lipid droplets in cholesterolosis of the gallbladder by increasing ABCA1 and NCEH1 expression. Molecular and Cellular Biochemistry 399: 7–15. [Google Scholar] [PubMed]
Ward NC, Watts GF, Eckel RH (2019). Statin toxicity. Circulation Research 124: 328–350. [Google Scholar] [PubMed]
Watson CE, Weissbach N, Kjems L, Ayalasomayajula S, Zhang Y et al. (2011). Treatment of patients with cardiovascular disease with L-4F, an apo-A1 mimetic, did not improve select biomarkers of HDL function. Journal of Lipid Research 52: 361–373. [Google Scholar] [PubMed]
Weinreich M, Frishman WH (2014). Antihyperlipidemic therapies targeting PCSK9. Cardiology Reviews 22: 140–146. [Google Scholar]
Wolska A, Reimund M, Sviridov DO, Amar MJ, Remaley AT (2021). Apolipoprotein mimetic peptides: Potential new therapies for cardiovascular diseases. Cells 10: 597. [Google Scholar] [PubMed]
Wu H, Feng K, Zhang C, Zhang H, Zhang J, Hua Y, Dong Z, Zhu Y, Yang S, Ma C (2021). Metformin attenuates atherosclerosis and plaque vulnerability by upregulating KLF2-mediated autophagy in apoE-/- mice. Biochemical and Biophysical Research Communications 557: 334–341. [Google Scholar] [PubMed]
Wu Y, Li X, Guo Y, Jia Y, Sun P (2022). Pravastatin reduces matrix metalloproteinases expression and promotes cholesterol efflux in osteoarthritis chondrocytes. Evidence-Based Complementary and Alternative Medicine 2022: 9666963. [Google Scholar] [PubMed]
Wu ZH, Zhao SP (2009). Niacin promotes cholesterol efflux through stimulation of the PPARgamma-LXRalpha-ABCA1 pathway in 3T3-L1 adipocytes. Pharmacology 84: 282–287. [Google Scholar] [PubMed]
Wygrecka M, Alexopoulos I, Potaczek DP, Schaefer L (2023). Diverse functions of apolipoprotein A-I in lung fibrosis. The American Journal of Physiology-Cell Physiology 324: C438–C446. [Google Scholar] [PubMed]
Wójcicka G, Jamroz-Wiśniewska A, Czechowska G, Korolczuk A, Marciniak S, Bełtowski J (2016). The paraoxonase 1 (PON1platelet-activating factor acetylohydrolase (PAF-AH) and dimethylarginine dimethylaminohydrolase (DDAH) activity in the metformin treated normal and diabetic rats. European Journal of Pharmacology 789: 187–194. [Google Scholar]
Xing H, Liang C, Wang C, Xu X, Hu Y, Qiu B (2022). Metformin mitigates cholesterol accumulation via the AMPK/SIRT1 pathway to protect osteoarthritis chondrocytes. Biochemical and Biophysical Research Communications 632: 113–121. [Google Scholar] [PubMed]
Yahya R, Favari E, Calabresi L, Verhoeven AJM, Zimetti F et al. (2016). Lomitapide affects HDL composition and function. Atherosclerosis 251: 15–18. [Google Scholar] [PubMed]
Yang J, Chen Y, Zou T, Xue B, Yang F et al. (2023). Cholesterol homeostasis regulated by ABCA1 is critical for retinal ganglion cell survival. Science China Life Sciences 66: 211–225. [Google Scholar] [PubMed]
Yang K, Wang J, Xiang H, Ding P, Wu T, Ji G (2022a). LCAT- targeted therapies: Progress, failures and future. Biomedicine & Pharmacotherapy 147: 112677. [Google Scholar]
Yang N, Yang Y, Huang Z, Chen HW (2022b). Deregulation of cholesterol homeostasis by a nuclear hormone receptor crosstalk in advanced prostate cancer. Cancers 14: 3110. [Google Scholar] [PubMed]
Ye G, Chen G, Gao H, Lin Y, Liao X et al. (2019). Resveratrol inhibits lipid accumulation in the intestine of atherosclerotic mice and macrophages. Journal of Cellular and Molecular Medicine 23: 4313–4325. [Google Scholar] [PubMed]
Ying Q, Ronca A, Chan DC, Pang J, Favari E, Watts GF (2022). Effect of a PCSK9 inhibitor and a statin on cholesterol efflux capacity: A limitation of current cholesterol-lowering treatments? European Journal of Clinical Investigation 52: e13766. [Google Scholar] [PubMed]
Yoon M (2009). The role of PPARalpha in lipid metabolism and obesity: Focusing on the effects of estrogen on PPARα actions. Pharmacological Research 60: 151–159. [Google Scholar] [PubMed]
Yuan W, Ernst K, Kuai R, Morin EE, Yu M et al. (2023). Systematic evaluation of the effect of different apolipoprotein A-I mimetic peptides on the performance of synthetic high-density lipoproteins in vitro and in vivo. Nanomedicine 48: 102646. [Google Scholar] [PubMed]
Yvan-Charvet L, Kling J, Pagler T, Li H, Hubbard B, Fisher T, Sparrow CP, Taggart AK, Tall AR (2010). Cholesterol efflux potential and antiinflammatory properties of high-density lipoprotein after treatment with niacin or anacetrapib. Arteriosclerosis, Thrombosis and Vascular Biology 30: 1430–1438. [Google Scholar] [PubMed]
Zanoni P, Khetarpal SA, Larach DB, Hancock-Cerutti WF, Millar JS et al. (2016). Rare variant in scavenger receptor BI raises HDL cholesterol and increases risk of coronary heart disease. Science 351: 1166–1171. [Google Scholar] [PubMed]
Zhang M, Lei D, Peng B, Yang M, Zhang L, Charles MA, Rye KA, Krauss RM, Johns DG, Ren G (2017). Assessing the mechanisms of cholesteryl ester transfer protein inhibitors. Biochimica et Biophysica Acta (BBA)—Molecular and Cell Biology of Lipids 1862: 1606–1617. [Google Scholar] [PubMed]
Zhang X, Qin Y, Wan X, Liu H, Lv C, Ruan W, He L, Lu L, Guo X (2021). Rosuvastatin exerts anti-atherosclerotic effects by improving macrophage-related foam cell formation and polarization conversion via mediating autophagic activities. Journal of Translational Medicine 19: 62. [Google Scholar] [PubMed]
Zheng S, Du Y, Ye Q, Zha K, Feng J (2021). Atorvastatin enhances foam cell lipophagy and promotes cholesterol efflux through the AMP-activated protein kinase/mammalian target of rapamycin pathway. Journal of Cardiovascular Pharmacology 77: 508–518. [Google Scholar] [PubMed]
Zhu L, Luu T, Emfinger CH, Parks BA, Shi J et al. (2018). CETP inhibition improves HDL function but leads to fatty liver and insulin resistance in CETP-expressing transgenic mice on a high-fat diet. Diabetes 67: 2494–2506. [Google Scholar] [PubMed]
Zineh I (2007). Pharmacogenetics of response to statins. Current Atherosclerosis Reports 9: 187–194. [Google Scholar] [PubMed]
Cite This Article
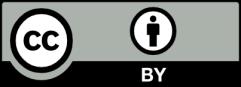
This work is licensed under a Creative Commons Attribution 4.0 International License , which permits unrestricted use, distribution, and reproduction in any medium, provided the original work is properly cited.