Open Access
REVIEW
Mannose metabolism and immune regulation: Insights into its therapeutic potential in immunology-related diseases
School of Life Sciences, Changchun Normal University, Changchun, 130032, China
* Corresponding Author: KAI SONG. Email:
BIOCELL 2023, 47(11), 2535-2546. https://doi.org/10.32604/biocell.2023.030781
Received 31 July 2023; Accepted 14 September 2023; Issue published 27 November 2023
Abstract
Mannose, a different isomer of the hydroxyl group at the C-2 position of glucose, shares the same transport carrier protein with glucose to enter cells and participate in the regulation of glucose metabolism. It affects cell growth, differentiation, and function and plays an active role in tumor immunity and inflammatory processes. This paper provides theoretical support for expanding the clinical applications of mannose by exploring its constitution, metabolic pathways, and role in regulating immune cell function and treating immunology-related diseases.Graphic Abstract
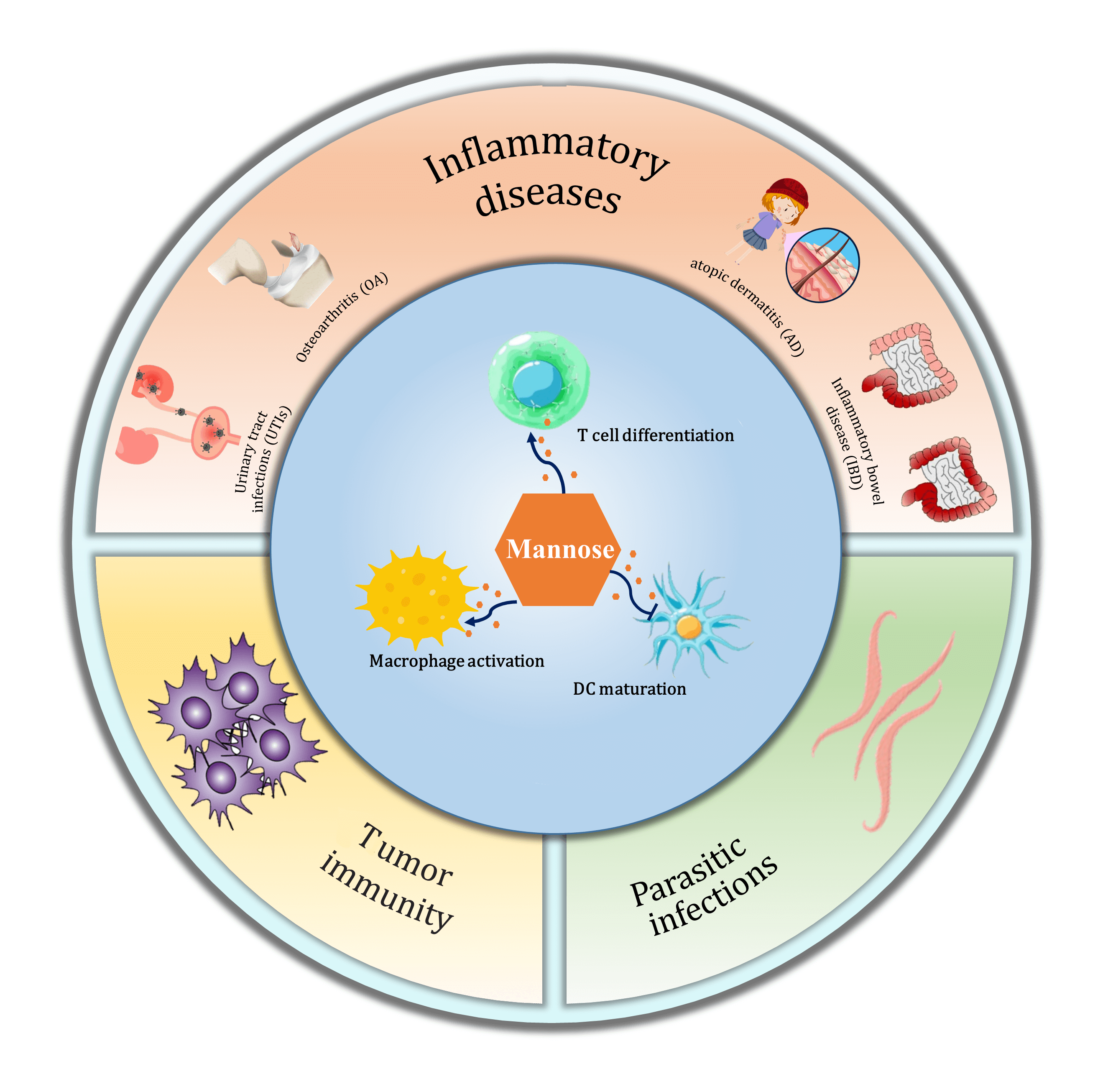
Keywords
Mannose, a simple six-carbon sugar, is structurally similar to glucose—an epimer of the C-2 hydroxyl of glucose—and is naturally abundant, as depicted in Fig. 1 (Hu et al., 2016). Mannose is an essential component of glycoconjugates found in body fluids and tissue fluids, such as serum globulins and cell surface mannans. In normal human blood, the mannose concentration is less than one-fiftieth of the glucose concentration, which is approximately 50–100 μM (Alton et al., 1998; Sharma et al., 2014). Mannose is abundant in many plants and fruits; for example, mannose is most abundant in Mango lingonberry. Palm kernels, coffee grounds, and date powder are better raw materials for mannose production. Mannans, found in microbial cell walls, are used for mannose extraction (Herman, 1971; Wu et al., 2019).
Figure 1: Chemical structures of D-mannose and D-glucose, adapted from Hu et al. (2016).
Mannose is often used as a food additive in the production of food because of its non-toxic, strong sweetness, and low caloric properties. Moreover, mannose has essential physiological functions in human metabolism and disease improvement, such as promoting the balance of intestinal flora and insulin secretion. It can also be used as a component of vitamin synthesis, anti-tumor, and immunomodulatory drugs, thereby attracting increasing research interest. In this paper, we review the research on the metabolic regulation of mannose in regulating immune cell function and immune-related diseases to provide ideas for understanding the relationship between metabolic regulation and immune cell function and expanding the clinical applications of mannose.
Mannose and glucose share the same transport protein (GLUT) to enter the cell and regulate glucose metabolism (Sharma and Freeze, 2011; Mueckler and Thorens, 2013). Similar to glucose metabolism, mannose entering the body is initially catalyzed by hexokinase (HK) to form mannose-6-phosphate (M-6-P), followed by phosphomannomutase (PMM2) to form mannose-1-phosphate (M-1-P). M-1-P is ultimately converted to guanosine diphosphate mannose (M-GDP)—a mannose donor activated by the glycosylation modification center—that contributes to the protein glycosylation pathway by binding mannose to glycoproteins and glycolipids. M-6-P, an intermediate metabolite of mannose, can also be catalyzed by phosphomannose isomerase (PMI) to form fructose-6-phosphate (F-6-P), which regulates the glucose metabolic pathway and influences the glucose glycolytic pathway, as depicted in Fig. 2 (Sharma and Freeze, 2011; Sharma et al., 2014). The accumulation of M-6-P within the cell inhibits the glycolytic pathway. PMI catalyzes the reversible conversion of M-6-P to F-6-P, establishing a link between mannose and other metabolic pathways. The fate of M-6-P is mainly dependent on the ratio of intracellular PMI to PMM2, and the greater the ratio of intracellular PMI activity to PMM2 activity, the more favorable the conditions are for the production of F-6-P, and conversely, the more favorable conditions are for the N-glycosylation pathway (Sharma and Freeze, 2011; Sharma et al., 2014).
Figure 2: Mannose metabolic pathway in cells. HK, hexokinase; PMI, phosphomannose isomerase; PMM2, phosphomannomutase; PGI, phosphoglucose isomerase; PFK, phosphofructokinase; PKM, Pyruvate Kinase M; LDH, lactate dehydrogenase; GFAT, 6-phosphate fructose aminotransferase; OGT, O-GlcNAc transferase; M-6-P, mannose-6-phosphate; M-1-P, mannose-1-phosphate; G-6-P, glucose-6-phosphate; F-6-P, fructose-6-phosphate; F-1,6-2P, fructose-1,6-diphosphate; GlcN-6-P, glucosamine-6-phosphate; M-GDP, guanosine diphosphatemannose; PPP, pentose phosphate pathway; HBP, hexosamine biosynthesis pathway.
Mannose Metabolism in Immune Cells
As the body’s primary immune response executors, immune cells play a crucial role in pathogen defense and immune homeostasis. Different immune cells manifest distinct metabolic characteristics when performing their immune functions at various stages of differentiation and development (Hu et al., 2022). During activation, dendritic cells (DCs) and T cells endure changes in their glucose and fatty acid metabolisms, and macrophage polarization is associated with energy and lipid metabolisms. The aerobic glycolytic pathway is the primary metabolic mode of inflammatory and rapidly proliferating immune cells; however, many non-inflammatory and non-rapidly proliferating immune cells, such as M2-type macrophages, Treg cells, and memory T cells, rely on the fatty acid-oxidation pathway (Pearce and Pearce, 2013; Olenchock et al., 2017). Many studies have revealed that immune cells rely on cellular metabolism to meet their energy and material requirements and that changes in metabolic pathways determine immune cell fate and their immune effector functions (Biswas, 2015).
Effect of mannose on the differentiation of Treg cells
Extensive research has focused on the function of glucose metabolism in immune cell maturation and differentiation, energy production, storage, and regulation. CD4+ T cells can be subdivided into several subpopulations, including Th1, Th2, Th9, Th17, Th22, and regulatory T (Treg) cells. Studies have confirmed the essential role of glucose in T cell activation and differentiation, demonstrating that upregulation of glycolytic flux is required for Th1, Th2, and Th17 differentiation (Wik and Skalhegg, 2022). However, the function of the glucose analog D-mannose in the immune response of T cells remains unknown. The role of D-mannose in T cell differentiation in a mouse model of diabetes and airway inflammation was first reported by Zhang et al. (2017). CFSE staining of mouse naive T cells stimulated with CD3/CD28 and mannose for 48–72 h demonstrated that mannose inhibited T cell proliferation. The mRNA expression levels of the cytokines Ifng, Il2, Il4, and Il10 associated with Th1 and Th2 decreased, whereas the levels of Foxp3 mRNA increased significantly. This finding suggests that mannose stimulates the development of Treg cells. D-mannose supplementation in drinking water suppressed immunopathological signs in mice with autoimmune diabetes and airway inflammation models and increased the proportion of Foxp3+ Treg cells in mice, as determined through in vivo experiments.
TGF-β signaling serves a crucial role in the production of Treg cells. Mannose-induced Treg cells exhibited similar inhibitory activity to TGF-β-induced Treg cells and inhibited the proliferation of naive T cells (Chen et al., 2003; Chen and Konkel, 2015; Zhang et al., 2017). The addition of mannose promoted TGF-β signaling in T cells, where the mRNA levels of Smad7 and Fos were increased markedly. An antibody that neutralizes TGF-β prevented TGF-β signaling and inhibited the mannose-induced increase in Foxp3 gene expression and Treg cells. For naive T cells in Smad3-deficient mice, mannose stimulation significantly decreased Treg cells, suggesting that TGF-β plays an essential function in the mannose-induced production of Treg cells.
Studies have reported that integrin αvβ8 and reactive oxygen species (ROS) are implicated in the TGF-β-induced activation of immune cells (Amarnath et al., 2007; Worthington et al., 2015). Similarly, the mRNA expression levels of Itgav and Itgb8 were significantly elevated in mannose-stimulated naive T cells. The deletion of integrin αvβ8 significantly decreased the mannose-induced production of Treg cells. Mannose stimulation increased ROS production in T cells, and after inhibiting ROS activity, the number of mannose-induced Treg cells decreased significantly. In integrin αvβ8-deficient naive T cells stimulated with mannose and supplemented with NAC to neutralize ROS activity, Treg cells were decreased by 70%–80%. This finding indicates that integrin αvβ8 and ROS play independent and complementary functions in mannose-mediated TGF-β activation and Treg production. Furthermore, the efficient utilization of fatty acid oxidation by mannose-stimulated T cells may account for the high level of ROS production. The unrecognized immunomodulatory function of D-mannose may have clinical applications in immunopathology and should be promising for treating inflammatory responses.
Effect of mannose on IFN-γ secretion in Th1 cell
The balance of Th1 and Th2 cell differentiation plays an important regulatory and paracrine role in the body’s immune response against pathogens. Excessive Th1 cell activation and IFN-γ production is associated with the development of autoimmune diseases such as rheumatoid arthritis, multiple sclerosis and certain hypersensitivity diseases (Rogers and Croft, 1999). Glycolysis stimulates the proliferation of Th1 cells and the production of the cytokine IFN-γ in Th1 cells (Shi et al., 2011; Chang et al., 2013). By inhibiting hexokinase activity, 2DG—a competitive inhibitor of phosphoglucose isomerase—inhibits the metabolic flux of glycolysis. Through PMI, glycolysis establishes a vital link to mannose metabolism; therefore, it was hypothesized that mannose metabolism might also be involved in Th1 cell differentiation. Zygmunt et al. (2018) confirmed this hypothesis (Zygmunt et al., 2018).
Adding exogenous mannose to a glucose-containing medium could promote cell proliferation and compensate for the decreased IFN-γ in Th1 cells caused by 2DG and galactose. However, neither the inhibitory effect on cell motility caused by a reduction in glycolytic metabolism nor lactate production was affected. In terms of promoting IFN-γ cell production, the addition of mannose alone to the Th1 differentiation medium had the same effect as the addition of glucose alone. The balance of hexose metabolism is required for expression in Th1 cells, and the concentration of hexose in the medium regulates the expression of IFN-γ and the proliferation of Th1 cells. Compared with 10 or 25 mM glucose culture conditions, IFN-γ expression was inhibited in 1 mM glucose culture conditions. Low concentrations of mannose (1 mM) promote IFN-γ expression and Th1 cell proliferation, whereas high concentrations (25 mM) exhibited the opposite effect. When glycolysis was inhibited by the addition of 2DG to Th1 cell cultures, the phosphorylation of T-bet, STAT1, and STAT4 governed the promotion of IFN-γ secretion by supplemental mannose in Th1 cells. When glycolysis was inhibited, phosphorylation of T-bet and STAT1, STAT4 in Th1 cells exhibited low expression levels, and mannose supplementation increased their expression, indicating that mannose regulates Th1 cell differentiation at the transcriptional level (Zygmunt et al., 2018).
Effect of mannose on macrophage activation
Macrophages are the first line of defense in intrinsic immunity; they are present throughout the body, respond to different pathogen signals resulting in varying activation states, and play a crucial role in the development and progression of inflammation. Their functional plasticity is contingent on the modification of metabolic processes. Macrophage activation increases glucose and glutamine uptake rates, lactate production rates, and glycolytic levels, thereby promoting the LPS-induced production of the inflammatory cytokine IL-1β. Glycolysis swiftly generates adenosine triphosphate (ATP) and biosynthetic intermediates to support the effector functions of macrophages, including glucose that can enter the pentose phosphate pathway and promote the maintenance of an inflammatory state (Pearce and Pearce, 2013; O’Neill et al., 2016).
Through in vitro experiments, Torretta et al. (2020) demonstrated that mannose could dose-dependently inhibit LPS-induced IL-1β production in mouse bone marrow-derived macrophages (BMDMs), thereby inhibiting macrophage activation (Torretta et al., 2020). The mannose receptor (MR)—a C-type lectin receptor expressed on phagocytes—binds mannose residues on pathogenic microorganisms and regulates inflammatory responses (Taylor et al., 2005). Exogenous mannose can enter the cell via GLUT to regulate glucose metabolism and bind to MR on the cell surface to influence cell function. By contrast, silencing MR expression in macrophages derived from mouse bone marrow did not affect the expression level of IL-1β inhibited by mannose, indicating that mannose inhibits IL-1β production in macrophages primarily via a pathway independent of MR. Intriguingly, the inhibitory effect of mannose on macrophage IL-1β was higher in low glucose (5.5 mM) culture conditions than in high grape content culture conditions, indicating that the effect of mannose on macrophage IL-1β secretion could be because of interference with the glucose metabolic pathway in cells. This hypothesis was confirmed through experiments, such as liquid chromatography-mass spectrometry analysis. In LPS-induced macrophages, the addition of mannose inhibited the rate of glucose consumption and lactate secretion and decreased the level of glucose-6-phosphate, an intermediate product of glucose metabolism. Furthermore, M-6-P levels increased within the cells. Inflammatory macrophages utilize less glucose in glycolysis after adding mannose; consequently, less carbon enters the TCA cycle, and the concentration of its intermediate product succinate decreases. This modification inhibits succinate-mediated activation of hypoxia-inducible factor 1A (HIF-1α), which sustains IL-1β production by promoting HIF-1α stabilization in pro-inflammatory macrophages (Tannahill et al., 2013). In healthy cells, PMI catalyzes the conversion of M-6-P to F-6-P, which then enters the glycolytic pathway. Overexpression of PMI in macrophages decreased the accumulation of M-6-P in LPS-induced pro-inflammatory macrophages under mannose stimulation. Increased PMI levels eliminated the effect of mannose on glucose utilization in LPS-activated BMDM and reversed the decrease in succinate and HIF-1α expression under mannose stimulation. The suppression of PMI expression and the increase in lactate content, succinate content, and IL-1β expression in LPS-stimulated macrophages treated with mannose indicates that PMI plays an essential role in the pro-inflammatory macrophage response to mannose.
The release of inflammatory mediators by activated macrophages plays a crucial role in pathogen immunity, but prolonged inflammatory stimulation is detrimental to tissue health, as seen in ulcerative colitis. In ulcerative colitis, mannose substantially mitigated the weight loss and inflammatory progression induced by dextran sodium sulfate (DSS) (Torretta et al., 2020). CD11b+ cells accumulated in the lamina propria of DSS-treated mice, and magnetic bead isolation of CD11b+ cells from the submucosa of the colon of DSS-treated mice was similar to that of inflammatory macrophages under LPS treatment in vitro, and mannose stimulation similarly attenuated their lactate and succinate levels and reduced their IL-1β level expression. The metabolic process of macrophage activation drives the safe and effective use of mannose as an anti-inflammatory agent.
Effect of mannose on dendritic cell function
In 1973, Steinman identified a unique cell type in the peripheral lymphoid organs of mice that exhibited numerous dendritic or pseudopod-like projections, leading to its designation as DCs (Steinman and Cohn, 1973). DCs are now recognized as the most potent antigen-presenting cells (APCs) and play a pivotal role in initiating and sustaining immune responses, particularly the T-cell-mediated immune response. Immature DCs in peripheral tissues capture and internalize antigens, which are then processed into MHC–peptide complexes. Subsequently, DCs migrate to secondary lymphoid organs, undergo maturation, and present the MHC-antigen peptide complexes to naive T cells, thus instigating an adaptive immune response (Banchereau and Steinman, 1998). Wang et al. (2021) investigated the role of mannose in autoimmune disease treatments and discovered that mannose’s immunomodulatory effects were tied to the induction of immature conventional dendritic cells (cDCs) and a reduction in effector T cell activation (Wang et al., 2021). The expression of surface molecules on DCs is dynamic, varying according to tissue localization and maturation status. Immature DCs predominantly express antigen-uptake-related molecules, such as CD32b and CD64, along with mannose receptors (MR, also known as CD206), which serve as endocytic receptors (Schuette et al., 2016; Cummings, 2022). Studies have indicated that mannose augmented CD206 expression on bone marrow-derived dendritic cells (BMDCs) in vitro and within chronic graft-vs.-host disease (cGVHD) and lupus-susceptible (B6/lpr) models in vivo. Moreover, CD32b and CD64 expression in mannose-treated BMDCs persisted at elevated levels after LPS stimulation, implying that mannose curtails DC maturation. In the cGVHD model, mannose administration reduced both inflammatory cytokine production and the expression of activation markers CD40 and CD80, subsequently inhibiting DC maturation. Concurrently, in a co-culture assay, D-mannose suppressed the antigen-specific proliferation and activation of CD4+ T cells (Wang et al., 2021).
Mannose metabolism in erythrocytes
In addition to transporting oxygen, erythrocytes—the most abundant type of cell in the blood—promote phagocytosis, eliminate immune complexes, and conduct immunomodulation. When human erythrocytes are incubated with mannose, M-6-P accumulates swiftly. PMI, not hexokinase, is the limiting enzyme for converting mannose to fructose in erythrocytes. PMI is also present in pig and rat erythrocytes. In hexokinase-deficient human cells, glucose utilization was decreased, whereas mannose utilization was elevated, and PMI activity was significantly increased. Hexokinase-deficient cells incubated with mannose accumulated M-6-P at a rate roughly a third of that of normal cells. Hexokinase-deficient cells preferentially utilize mannose over glucose more rapidly because of PMI’s regulatory effect on mannose utilization (Beutler and Teeple, 1969).
Erythrocytes can be stored under conventional conditions for up to 42 days, and the process that degrades their quality and impairs their oxygen transport function during storage is known as erythrocyte storage injury (RSL). It is possible to reduce RSL to manipulate the metabolic processes of erythrocytes and optimize the components in the erythrocyte storage solution. Erythrocytes stored at 9 or 18 mmol/L in a storage solution SAGM containing uniformly labeled 13C-fructose or 13C-mannose absorbed fructose and mannose in the presence of glucose when refrigerated. Mannose was more efficiently metabolized via glycolysis than glucose. Fructose decreased ATP levels and made a negligible contribution to ATP maintenance. By contrast, the contribution to ATP for SAGM with added mannose was essentially the same as for SAGM without added mannose. After 10 days of storage, adding fructose or mannose to erythrocyte SAGM concentrates may not counteract the alterations in erythrocyte metabolism. At high glucose concentrations, neither fructose nor mannose enhanced the ability of SAGM to maintain and protect erythrocyte mass during storage (Rolfsson et al., 2017).
Mannose Metabolism in Immune-Related Diseases
Role of mannose in tumor immunity
In the early 20th century, Prof. Otto Warburg made the pivotal discovery that tumor cells preferentially metabolize glucose to lactic acid via glycolysis, even in the presence of ample oxygen. This phenomenon, termed the ‘Warburg effect’, spurred subsequent research into tumor metabolism (Warburg, 1956). Indeed, metabolic reprogramming is now recognized as a critical hallmark of cancer (Hanahan, 2022). In terms of the oxidative phosphorylation metabolism required to sustain cellular life processes, tumor cells are wholly distinct from normal cells. A series of metabolic alterations in tumor cells, mainly characterized by the Warburg effect, exhibit large amounts of glucose uptake, enhanced aerobic glycolytic metabolism, and production of large quantities of lactic acid, which are conducive to malignant proliferation, invasive metastasis, and adaptation to a hostile survival environment of tumors. Therefore, studies have focused on modulating glucose metabolic pathways to treat tumors (Vander Heiden et al., 2009; Koppenol et al., 2011; Pavlova and Thompson, 2016). In 2018, Kevin M. Ryan’s team at Cancer Research UK demonstrated that mannose inhibits tumor growth by interfering with cellular glucose metabolism and substantially boosts the anti-tumor effects of chemotherapy drugs (Cisplatin and Adriamycin). Only mannose significantly inhibited the growth of human osteogenic sarcoma cell lines U2OS-E1a and Saos-2 and human pancreatic cancer cell line KP-4 when treated with high concentrations (25 mM) of each of the five monosaccharides (mannose, glucose, galactose, fructose, and fucose). When mannose enters tumor cells, it accumulates intracellularly as M-6-P, which inhibits tumor cell proliferation by interfering with glucose metabolism and blocking the tumor’s energy source. This study also revealed a correlation between the level of PMI expression in tumor cells and the inhibition of tumor cell growth by mannose. Tumor cells with low levels of PMI expression or reduced function were more sensitive to mannose therapy. By contrast, mannose had no significant inhibitory effect or only a weak effect on the growth of tumor cells containing higher PMI levels by interfering with PMI expression via RNA interference, thereby rendering the cells sensitive (Gonzalez et al., 2018).
Zhong et al. (2022) obtained comparable findings that regarding the role of mannose in advanced prostate cancer (PCa). Mannose inhibits PCa cell proliferation and promotes apoptosis. The process is regulated by altered mitochondrial mechanisms. Intracellular accumulation of mannose decreased mitochondrial membrane potential, leading to decreased ATP production in PCa cells, increased ROS levels in PCa cells, and enhanced expression of the pro-apoptotic factors Bax and Bak. Mannose-treated PCa cells altered mitochondrial morphology and diminished the mitochondrial fission protein FIS1. Furthermore, low expression of PMI—a key enzyme of mannose metabolism—was associated with a poor prognosis in PCa patients, whereas downregulation of PMI expression in PCa cells increased the anticancer effect of mannose (Zhong et al., 2022). Cancer metabolism is temporally and spatially heterogeneous. The metabolic phenotype of tumor cells is not uniform across cancer types and undergoes continuous change during tumor progression (Xiao et al., 2023b). The signaling pathways used to treat tumors must vary because of the heterogeneity of cancer cells. Mannose inhibits proliferation and promotes apoptosis in glioma cells, and PMI inhibition facilitates apoptosis in glioma cells. Most processes inhibit the Wnt/β-catenin signaling pathway. Mannose inhibits the expression of 6-methylguanine DNA methyltransferase (MGMT) to increase glioma cells’ sensitivity to temozolomide (TMZ) (Fei et al., 2022). PMI depletion decreased the phosphorylation of FGFR family receptors in malignant glioma cell lines and the signaling of FRS2, Akt, and MAPK. PMI knockdown substantially decreased the clonal survival of glioma cells following ionizing radiation (Cazet et al., 2014).
Triple-negative breast carcinomatosis (TNBC)—characterized by cells lacking estrogen receptor (ER), progesterone receptor (PR), and human epidermal growth factor receptor 2 (HER2)—is the least prognostic and most aggressive molecular subtype of breast tumors, and chemotherapy and radiotherapy are less effective treatments for TNBC (Harbeck et al., 2019; Yin et al., 2020). Zhang et al. (2022) revealed a new function and mechanism for mannose in TNBC immunotherapy and radiation therapy (Zhang et al., 2022). Mannose facilitates the degradation of PD-L1 by affecting its glycosylation, thereby enhancing the efficacy of immunotherapy and radiotherapy in TNBC. PD-L1 is a classic tumor immune checkpoint molecule that inhibits the proliferation and cytotoxicity of T cells by binding to PD1 molecules on T cells, thereby facilitating tumor cell evasion of T cell surveillance (Freeman et al., 2000). Mannose inhibits PD-L1 expression by interfering with PD-L1 glycosylation. AMPK-mediated phosphorylation of the PD-L1 S195 site promotes PD-L1 ubiquitination and targets it to the proteasome. The degradation of PD-L1 by mannose promotes T-cell activation and the destruction of tumor cells by T-cells. The combination of mannose and PD-L1 blockers substantially inhibited the growth of TNBC and increased the survival rate of mice with tumors (Zhang et al., 2022). In addition, PD-L1 can bind to RNA in the cytoplasm and protect the mRNA stability of genes involved in DNA damage repair, promoting DNA damage repair in tumor cells (Tu et al., 2019). Mannose-induced PD-L1 degradation also destabilizes messenger RNA of DNA damage-repair-related genes, which sensitizes breast cancer cells to ionizing radiation therapy and promotes radiation therapy for TNBC in rodents (Zhang et al., 2022). It established a relationship between cellular metabolism and tumor immune evasion, revealed the mechanism of D-mannose targeting PD-L1 degradation, and proposed a novel clinical treatment strategy for TNBC.
Previous studies on mannose for tumor cell proliferation and metabolism have demonstrated that mannose has a beneficial effect on treating low PMI-expressing tumors. By contrast, hematological malignancies and leukemia cells express high levels of PMI. PMI enables mannose to enter the glycolytic pathway, TCA cycle, and pentose phosphate pathway (PPP) of leukemia cells, providing an energy source during glucose deprivation. Therefore, mannose in the blood can be considered an energy source for the glycolytic pathway. However, when mannose levels exceed the PMI-treated load, PMI expression is inhibited, leading to the accumulation of M-6-P caused by mannose load, which can inhibit glycolytic levels, mitochondrial metabolism, or the expression of related genes in the TCA cycle, thereby inhibiting the growth of leukemic cells, as shown in Fig. 3 (Saito et al., 2021). In acute myeloid leukemia (AML), high PMI expression is a negative prognostic factor. Meanwhile, AML cells increased expression of genes related to oxidative phosphorylation and fatty acid metabolism. Research has shown that inhibiting PMI, the initial enzyme in the mannose metabolism pathway, enhances the sensitivity to both cytarabine and FLT3 inhibitors across various AML models. This PMI inhibition activates the ATF6 component of the unfolded protein response (UPR). Subsequently, this component impairs fatty acid metabolism, resulting in the intracellular accumulation of polyunsaturated fatty acids (PUFA), lipid peroxidation, and the induction of ferroptotic cell death in AML cells (Woodley et al., 2023). These findings underscore the significance of metabolic reprogramming in AML treatment and the potential benefits of combining PMI inhibition with mannose supplementation in leukemia therapy.
Figure 3: Relationship between mannose loading and PMI expression in metabolic regulation. The figure adapted from Saito et al. (2021).
Pyroptosis, a recently identified form of regulated cell death, is characterized by perforations in the cell membrane. It is executed by members of the gasdermin family, including GSDMA, GSDMB, GSDMC, GSDMD, and GSDME (Kovacs and Miao, 2017). Notably, GSDME is predominantly expressed in the intestine and kidney, whereas its expression remains low in most tumor cells (Wang et al., 2017). Particularly during cancer chemotherapy, GSDME-mediated pyroptosis in tissue cells is linked to chemotherapy-induced side effects, intensifying patients’ distress and influencing the treatment trajectory (Shen et al., 2021; Xia et al., 2021). Ai et al. (2023) evidenced that mannose counteracts GSDME-mediated pyroptosis via AMPK activation, triggered by the metabolite N-acetylglucosamine-6-phosphate (GlcNAc-6P) (Ai et al., 2023). Intracellular entry of mannose elevates GlcNAc-6P levels via the HBP pathway. This metabolite subsequently binds to AMPK, promoting its phosphorylation by LKB1. The activated AMPK then phosphorylates the Thr6 site of GSDME, preventing caspase-3 binding and cleavage, thereby inhibiting pyroptosis. Beyond uncovering mannose’s novel mechanism in pyroptosis inhibition, this research also substantiates that mannose can mitigate intestinal toxic side effects caused by chemotherapeutic agents, such as cisplatin or oxaliplatin, both in mouse models and clinical patients with gastrointestinal cancers. Such insights breathe new life into an old drug, offering fresh perspectives and strategies for clinically alleviating chemotherapy’s side effects and holding promising therapeutic potential.
Role of mannose metabolism in inflammatory diseases
The effect of mannose on inflammatory diseases has been continuously discovered through extensive research on mannose. Urinary tract infections (UTIs) triggered by bacterial invasion of the urinary tract epithelium, which is most common in women, have a high recurrence rate, and their incidence increases significantly after menopause (Foxman and Brown, 2003). The ability of lectin molecules in the bacterial cell wall to bind to structures, such as mannose and fucose, on the surface of human cells is a significant factor in infection development. Escherichia coli, specifically Uropathogenic E. coli (UPEC), is the primary causative agent of symptomatic UTIs, contributing to approximately 80% of all UTIs. The type I bacterial pilus adhesin protein, FimH, found in UPEC binds to mannose structures on urinary tract epithelial cells. This binding facilitates bacterial adhesion and elicits an inflammatory response (Hatton et al., 2020; Krogfelt et al., 1990). Antibiotics are the first line of defense in the treatment of UTIs. However, the escalating challenge of antibiotic resistance underscores the imperative need for non-antibiotic alternatives in UTI prevention and treatment. Numerous studies have validated the substantial benefits of mannose in alleviating urinary tract infections and enhancing patients’ quality of life. The mechanism is that mannose inhibits bacterial adhesion to the urinary epithelium and simulates the barrier function of the uroepithelium, thereby effectively inhibiting UTIs. Bacteria bound to free D-mannose in the urine, as opposed to proteins on the surface of vascular cells, are expelled from the body via the urine stream (Kranjčec et al., 2013; Domenici et al., 2016; Cooper et al., 2022). Lenger et al. (2020) reported that mannose doses ranging from 420 mg to 2 g, administered 1 to 3 times daily over intervals of one week per month, were generally well tolerated. Side effects were minimal, with diarrhea reported by a small percentage of participants, and there was evidence of protection against recurrent UTIs (Lenger et al., 2020). In a separate study, Kyriakides et al. (2021) found that dosages of mannose between 500 mg to 2 g, given over varying durations (from 3 days up to 6 months), not only reduced the incidence of recurrent UTIs but also extended the intervals between successive infections, improving overall patient well-being (Kyriakides et al., 2021). Ongoing and optimized clinical trials continue to focus on the potential of mannose in UTI prevention and treatment (Konesan et al., 2022). Radulescu et al. (2020) highlighted that a combined treatment of cranberry extract and D-mannose might bolster the sensitivity of uropathogens to antibiotics during acute UTI treatment (Radulescu et al., 2020). Moreover, post-cystoscopy administration of D-mannose and Saccharomyces boulardii was found to markedly decrease UTI incidence, mitigate the severity of lower urinary tract symptoms (LUTS), and reduce localized discomfort (Quattrone et al., 2023). Lenger et al. (2023) further investigated the combined efficacy of mannose with vaginal estrogen therapy (VET) in preventing recurrent UTIs (Lenger et al., 2023).
Osteoarthritis (OA) is a complex disease characterized by cartilage degeneration, bone redundancy formation, joint inflammation, and subchondral bone remodeling, which has been challenging to treat because of its heterogeneity. IL-1β promotes matrix-degrading enzyme activity and apoptosis in chondrocytes, thereby accelerating the cartilage degeneration associated with osteoarthritis. Mannose has a protective effect on the cartilage in OA. D-mannose may induce autophagy in IL-1β-treated rat chondrocytes by promoting AMPK phosphorylation, inhibiting OA degeneration. In vivo experiments confirmed that a median dose of D-mannose inhibited monosodium iodoacetate (MIA)-induced OA development (Lin et al., 2021). Conversely, chondrocyte ferroptosis is implicated in the progression of OA. D-mannose can mitigate OA progression by suppressing HIF-2α-mediated sensitization of chondrocytes to ferroptosis. These findings hint at mannose’s potential as a therapeutic strategy for diseases linked to Ferroptosis (Zhou et al., 2021).
Mannose is linked to atopic dermatitis (AD), a chronic inflammatory disease with recurrent pruritus. In a mouse model of AD induced by DNCB (2,4-dinitrochlorobenzene), D-mannose substantially reduced skin damage and restored skin barrier function (Luo et al., 2022). D-mannose inhibited the TNF-α-induced increase in inflammatory cytokine expression and substantially downregulated the TNF-α-induced activation of the mTOR/NF-κB signaling pathway. This finding is the first indication that D-mannose plays a role in local skin inflammation, suggesting its potential use in treating AD (Luo et al., 2022). Psoriasis is a chronic autoimmune inflammatory skin condition with a yet-to-be-determined pathogenesis, posing significant treatment challenges. This condition places a significant physical and psychological toll on those afflicted. The pathogenesis of psoriatic inflammation involves a complex, self-perpetuating cycle driven by keratinocytes (KC) and various immune cells (Armstrong and Read, 2020). Recent findings have suggested that mannose can modulate this cycle. Specifically, mannose suppresses the expression of HIF-1α and diminishes the expression level of the chemokine CCL20 in keratinocytes. This activity, in turn, hampers the local recruitment of Th17 cells, disrupting the KC-Th17 cell feedback loop and consequently reducing the cutaneous inflammation characteristic of psoriasis in mouse models (Jiang et al., 2023).
Inflammatory bowel disease (IBD) is a chronic inflammatory condition with a rising incidence worldwide. It encompasses conditions such as Crohn’s disease (CD) and ulcerative colitis (UC). IBD is primarily characterized by immune response dysregulation, aberrant cytokine secretion, intestinal flora dysbiosis, and compromised barrier function (Maloy and Powrie, 2011). It is reported that patients with IBD and mice with experimental colitis had elevated mannose levels. In IL-10-deficient mice, mannose ameliorated the intestinal barrier damage caused by DSS-induced and spontaneous colitis. In the context of intestinal epithelial damage, mannose improved lysosomal integrity and limited the release of histone B, thereby preventing mitochondrial dysfunction and myosin light chain kinase (MLCK)-induced disruption of tight junctions. Mannose and mesalazine have a synergistic therapeutic effect on murine colitis (Dong et al., 2022). IBD is a multifaceted condition with a complex etiology involving susceptibility genes, immune system dynamics, environmental influences, and gut microbiota interactions (Ramos and Papadakis, 2019). At its core, IBD pathogenesis hinges on intricate epithelial-immune interactions. A compromised intestinal epithelial function allows gut microbes to infiltrate the lamina propria, activating intestinal immune cells. This cascade produces an influx of inflammatory cytokines, further damaging intestinal epithelial cells (IECs). Notably, excessive endoplasmic reticulum stress (ERS)—a distinctive feature of IBD pathogenesis—results in IEC apoptosis, further compromising epithelial integrity (Eugene et al., 2020). Xiao et al. (2023a) unveiled a novel pathway wherein mannose metabolism mitigates colitis development and aids in its recovery (Xiao et al., 2023a). In this context, macrophages release the inflammatory cytokine TNF-α, exacerbating pathological ERS in IECs. Mannose plays a dual protective role. First, mannose counteracts this stress by ensuring consistent protein N-glycosylation, curtailing inflammatory responses in colonic macrophages and preserving epithelial cell integrity. Second, mannose curbs TNF-α mRNA translation by reducing 3-phosphoglyceraldehyde levels in macrophages, subsequently fostering GAPDH’s binding affinity to TNF-α mRNA. Therefore, mannose metabolism effectively disrupts the detrimental interplay between TNF-α-afflicted IECs and intestinal macrophages, rectifying the perturbed immune metabolism within the gastrointestinal tract. Further insights reveal marked disparities in mannose metabolism-associated enzymes in the intestinal mucosa of IBD patients compared with those of healthy individuals, indicating dysregulation of mannose metabolism in IBD-afflicted colonic mucosa. Specifically, PMM2 and PMI emerge as pivotal mannose-metabolizing enzymes. A deep dive into single-cell sequencing datasets unveiled that IECs specifically upregulate PMM2 to alleviate ERS, whereas colon macrophages predominantly downregulate PMI. This differential expression implies that as IECs necessitate elevated PMM2 expression to counteract ERS, lamina propria’s immune cells, such as macrophages, demand glycolysis down-regulation to temper their inflammatory response. In sum, these revelations underscore the closely-knit anti-ERS and anti-inflammatory attributes of mannose, which collectively fortify the intestinal barrier against colitis.
Role of D-mannose in parasitic infections
Not only does D-mannose play a role in the treatment of tumors and inflammatory diseases, but it has also been reported to suppress Plasmodium infection by modulating multiple host immune pathways. Plasmodium berghei-infected mice fed D-mannose experienced weight loss and a reduction in parasitemia without significant side effects, a mechanism that is independent of cellular oxidative stress. Mannose increases the accumulation of splenic macrophages in P. berghei-infected C57BL/6 mice. Mannose reduced the incidence of experimental cerebral malaria (ECM) in a rodent disease model. Mannose treatment of P. berghei-infected mice decreased the expression of the T-cell activation marker CD69 in peripheral blood and brain, the expression of mRNA levels of the inflammatory factors IFN-γ and TNF-α, and the expression of CXCR3, which prevented the migration of activated T cells to the brain. These findings suggest that mannose could be used as a potential malaria treatment aid (Lv et al., 2022).
Sugar is not only associated with flavor but also serves as a fundamental biomolecule, acting as the primary energy source for all living organisms to fuel their vital functions. Beyond this energy role, it furnishes the carbon backbone for synthesizing proteins, nucleic acids, and lipids. It also plays a pivotal role in cell recognition and immunological processes. Glucose, a hexose monosaccharide with six carbon atoms, is a crucial component of a balanced diet, and consequently, the study of glucose metabolism has garnered significant attention. Disruptions in glucose metabolism pathways, including glycolysis, the pentose phosphate pathway (PPP), and the tricarboxylic acid (TCA) cycle, are considered critical in metabolic disorders and cancer progression (Chen et al., 2019; Bose et al., 2021). However, glucose is not the sole hexose metabolized by cells (Lieu et al., 2021). Mannose, another monosaccharide with a structure remarkably similar to glucose, has significant structural roles in various legumes, fruits, and plants, predominantly as mannans, hemicelluloses, and cellulose. On a cellular level, D-mannose and its derivatives play central roles in most glycosylation reactions (Hu et al., 2016). These reactions are essential for intricate cellular processes, including energy production and glycosylation (Alton et al., 1998). The metabolism of mannose is intricately connected to glucose metabolism through isomerization reactions, with the conversion of M6P to F6P, as PMI serves as a nexus between glucose and mannose metabolism (Hu et al., 2016). Intriguingly, mannose has demonstrated the potential to suppress wound-induced inflammation (Jokela et al., 2013) and prevent obesity induced by high-fat diets (Sharma et al., 2018). Mannose is crucial in antibacterial, anti-inflammatory, and anti-tumor medical research and has become a “signal sugar” with a wide range of potential functions and values. It is unparalleled among pharmaceuticals in terms of availability, safety, and lack of toxicity. Mannose enters the body to interfere with glucose metabolism pathways; therefore, it is possible to treat diseases corresponding to it by modulating and intervening in mannose metabolism using various methods.
Recent research has placed a greater emphasis on the relationship between immune cells and metabolism. Metabolic pathways, encompassing sugars, fats, and amino acids, are intrinsically linked to the viability and activation of immune cells (Jung et al., 2019). Proper metabolic regulation is vital for the physiological activity of immune cells. Conversely, understanding the dysregulation of immune metabolism is pivotal in pathological studies. Notably, the role of mannose in regulating immune cell function has provided new explanations for the occurrence and treatment of the previously mentioned immunology-related diseases. Overall, as shown in Fig. 4, D-mannose stimulates Treg cell differentiation in human and mouse cells by activating TGF-β, which is mediated by the upregulation of integrin αvβ8 and increased production of ROS from fatty acid oxidation (Zhang et al., 2017). Exogenous mannose supplementation can backfill the secretion of IFN-γ from Th1 cells when the glycolytic pathway is inhibited, a process regulated by phosphorylation of T-bet, STAT4, and STAT4 (Zygmunt et al., 2018). Mannose can inhibit LPS-induced IL-1 secretion in macrophages in a dose-dependent manner by interfering with the glucose metabolic pathway and by downregulating succinate-induced HIF-1α expression (Torretta et al., 2020). Activated metabolic processes in inflammatory cells, such as T cells and macrophages, provide direction for the safe and effective use of mannose in immunologically relevant diseases and the intervention of inflammatory responses. Recent research has indicated that during activation, DCs undergo metabolic reprogramming; specifically, the metabolic pathway transitions from oxidative phosphorylation to aerobic glycolysis (Krawczyk et al., 2010; Pearce and Everts, 2015). Concurrent with DC activation, there is an observed regulation in glucose uptake, complemented by a redirection of metabolic fluxes toward anabolic processes, notably fatty acid synthesis (Everts et al., 2014). This metabolic shift during DC activation supports the synthesis of essential biomolecules, laying the foundational groundwork for further DC activation. Although studies have reported mannose’s inhibitory effect on DC maturation within autoimmune disease frameworks, the exact influence of mannose on DCs’ metabolic regulation warrants more extensive investigation. Otherwise, it is unknown whether mannose affects the proliferation, differentiation, migration, or motility of other types of immune cells. Meanwhile, mannose sensitivity is negatively correlated with PMI, and the absence of PMI in rapidly proliferating cells inhibits the Warburg effect. PMI expression was highly variable across cell types; the role of PMI in the response of pro-inflammatory immune cells to mannose warrants further investigation. The interactions of cells in the immune microenvironment under the influence of mannose, the cross-linking of metabolic networks, and the signaling pathways involved in the inflammatory response must also be investigated further.
Figure 4: Mannose metabolism regulates immune cell function.
Notably, although mannose and glucose enter cells via common transporters implicated in metabolic regulation, no reports have indicated which of the 14 GLUT transporter proteins identified in humans transports mannose preferentially. Moreover, MR is extensively expressed on the cell membrane surface of immune cells, such as macrophages and DCs, and MR induces the uptake and delivery of pathogens containing mannose structures by these cells (van der Zande et al., 2021). When considering the regulation of mannose metabolism in immune cells and its effect on the therapeutic efficacy of related diseases, it is essential also to consider whether mannose enters the organism’s metabolism via MR.
Exogenous mannose has been observed to inhibit growth across a diverse range of tumor cells, encompassing osteosarcoma, breast cancer, prostate cancer, intestinal cancer, glioma, and leukemia. Furthermore, it enhances the sensitivity of these cancer cells to chemotherapeutic agents. Concurrently, D-mannose has demonstrated protective qualities against induced models of type I diabetes, airway inflammation, arthritis, dermatitis, and inflammatory bowel disease. These protective effects also extend to systemic autoimmune models. The inhibitory effect of exogenous mannose on the proliferation of tumor cells and the alleviating effect on inflammation-related diseases and parasitic infections is a surprising discovery demonstrating the breadth of glycobiology’s application. Specifically, innovation in the field of tumor immunotherapy has benefited a significant number of cancer patients. Tumor cells can indirectly compete with immune cells for glucose assimilation, such as through tumor escape, to inhibit the activity and function of immune cells. By contrast, various metabolites produced by tumor cells can also affect the function of immune cells to varying degrees. In addition to directly inhibiting the growth of tumor cells, mannose can promote the degradation of the immune brake molecule PD-L1 by targeting it in tumor cells, thereby increasing the killing effect of T cells on tumor cells (Zhang et al., 2022), indicating an essential role for mannose in regulating immune homeostasis and providing a novel strategy for ensuring the normal metabolism of immune cells in tumor immunotherapy. Understanding the effect of mannose metabolism on immune cells and its application in immunological diseases not only improves our understanding of the immune regulatory process in diseases but is also essential for developing metabolism-based approaches to enhance the efficacy of immunotherapy based on immune cells. Although the clinical utility of mannose in the prevention and mitigation of UTIs is fairly established, its application in other immunologically related diseases remains experimental. A recent study noted reduced chemotherapy side effects in eight patients with gastrointestinal cancers following mannose supplementation. However, the sample size of this clinical study is notably limited. There is still a long way to go before mannose can be utilized effectively and safely in clinical trials for disease treatment.
Acknowledgement: We thank Home for Researchers Editorial Team (www.home-for-researchers.com) for language editing service.
Funding Statement: This work was supported by Natural Science Foundation Project of Jilin Provincial Department of Science and Technology (YDZJ202301ZYTS348).
Author Contributions: The authors confirm contribution to the paper as follows: study conception and design: YF.X., XP. W., and K.S.; draft manuscript preparation: QP.B., and P.L. All authors reviewed the results and approved the final version of the manuscript.
Availability of Data and Materials: Not applicable.
Ethics Approval: Not applicable.
Conflicts of Interest: The authors declare no conflicts of interest to report regarding the present study.
References
Ai YL, Wang WJ, Liu FJ, Fang W, Chen HZ et al. (2023). Mannose antagonizes GSDME-mediated pyroptosis through AMPK activated by metabolite GlcNAc-6P. Cell Research 66: 1–19. https://doi.org/10.1038/s41422-023-00848-6 [Google Scholar] [PubMed] [CrossRef]
Alton G, Hasilik M, Niehues R, Panneerselvam K, Etchison JR, Fana F, Freeze HH (1998). Direct utilization of mannose for mammalian glycoprotein biosynthesis. Glycobiology 8: 285–295. https://doi.org/10.1093/glycob/8.3.285 [Google Scholar] [PubMed] [CrossRef]
Amarnath S, Dong L, Li J, Wu Y, Chen W (2007). Endogenous TGF-β activation by reactive oxygen species is key to Foxp3 induction in TCR-stimulated and HIV-1-infected human CD4+CD25− T cells. Retrovirology 4: 1–16. https://doi.org/10.1186/1742-4690-4-57 [Google Scholar] [PubMed] [CrossRef]
Armstrong AW, Read C (2020). Pathophysiology, clinical presentation, and treatment of psoriasis a review. JAMA 323: 1945–1960. https://doi.org/10.1001/jama.2020.4006 [Google Scholar] [PubMed] [CrossRef]
Banchereau J, Steinman RM (1998). Dendritic cells and the control of immunity. Nature 392: 245–252. https://doi.org/10.1038/32588 [Google Scholar] [PubMed] [CrossRef]
Beutler E, Teeple L (1969). Mannose metabolism in the human erythrocyte. The Journal of Clinical Investigation 48: 461–466. https://doi.org/10.1172/JCI106003 [Google Scholar] [PubMed] [CrossRef]
Biswas SK (2015). Metabolic reprogramming of immune cells in cancer progression. Immunity 43: 435–449. https://doi.org/10.1016/j.immuni.2015.09.001 [Google Scholar] [PubMed] [CrossRef]
Bose S, Zhang C, Le A (2021). Glucose metabolism in cancer: The Warburg effect and beyond. Advances in Experimental Medicine and Biology 1311: 3–15. https://doi.org/10.1007/978-3-030-65768-0_1 [Google Scholar] [PubMed] [CrossRef]
Cazet A, Charest J, Bennett DC, Sambrooks CL, Contessa JN (2014). Mannose phosphate isomerase regulates fibroblast growth factor receptor family signaling and glioma radiosensitivity. PLoS One 9: e110345. https://doi.org/10.1371/journal.pone.0110345 [Google Scholar] [PubMed] [CrossRef]
Chang CH, Curtis JD, MaggiJr LB, Faubert B, Villarino AV et al. (2013). Posttranscriptional control of T cell effector function by aerobic glycolysis. Cell 153: 1239–1251. https://doi.org/10.1016/j.cell.2013.05.016 [Google Scholar] [PubMed] [CrossRef]
Chen L, Chen XW, Huang X, Song BL, Wang Y, Wang YG (2019). Regulation of glucose and lipid metabolism in health and disease. Science China Life Sciences 62: 1420–1458. https://doi.org/10.1007/s11427-019-1563-3 [Google Scholar] [PubMed] [CrossRef]
Chen W, Jin W, Hardegen N, Lei KJ, Li L, Marinos N, McGrady G, Wahl SM (2003). Conversion of peripheral CD4+CD25− naive T cells to CD4+CD25+ regulatory T cells by TGF-β induction of transcription factor Foxp3. Journal of Experimental Medicine 198: 1875–1886. https://doi.org/10.1084/jem.20030152 [Google Scholar] [PubMed] [CrossRef]
Chen W, Konkel JE (2015). Development of thymic Foxp3+ regulatory T cells: TGF-β matters. European Journal of Immunology 45: 958–965. https://doi.org/10.1002/eji.201444999 [Google Scholar] [PubMed] [CrossRef]
Cooper TE, Teng C, Howell M, Teixeira-Pinto A, Jaure A, Wong G (2022). D-mannose for preventing and treating urinary tract infections. Cochrane Database of Systematic Reviews 8: 1–57. https://doi.org/10.1002/14651858.CD013608.pub2 [Google Scholar] [PubMed] [CrossRef]
Cummings RD (2022). The mannose receptor ligands and the macrophage glycome. Current Opinion in Structural Biology 75: 102394. https://doi.org/10.1016/j.sbi.2022.102394 [Google Scholar] [PubMed] [CrossRef]
Domenici L, Monti M, Bracchi C, Giorgini M, Colagiovanni V, Muzii L, Panici PB (2016). D-mannose: A promising support for acute urinary tract infections in women. A pilot study. European Review for Medical and Pharmacological Sciences 20: 2920–2925. [Google Scholar] [PubMed]
Dong L, Xie J, Wang Y, Jiang H, Chen K et al. (2022). Mannose ameliorates experimental colitis by protecting intestinal barrier integrity. Nature Communications 13: 4804. https://doi.org/10.1038/s41467-022-32505-8 [Google Scholar] [PubMed] [CrossRef]
Eugene SP, Reddy VS, Trinath J (2020). Endoplasmic reticulum stress and intestinal inflammation: A perilous union. Frontiers in Immunology 11: 543022. https://doi.org/10.3389/fimmu.2020.543022 [Google Scholar] [PubMed] [CrossRef]
Everts B, Amiel E, Huang SC, Smith AM, Chang CH et al. (2014). TLR-driven early glycolytic reprogramming via the kinases TBK1-IKK epsilon supports the anabolic demands of dendritic cell activation. Nature Immunology 15: 323–332. https://doi.org/10.1038/ni.2833 [Google Scholar] [PubMed] [CrossRef]
Fei YQ, Shi RT, Zhou YF, Wu JZ, Song Z (2022). Mannose inhibits proliferation and promotes apoptosis to enhance sensitivity of glioma cells to temozolomide through Wnt/β-catenin signaling pathway. Neurochemistry International 157: 105348. https://doi.org/10.1016/j.neuint.2022.105348 [Google Scholar] [PubMed] [CrossRef]
Foxman B, Brown P (2003). Epidemiology of urinary tract infections. Infectious Disease Clinics of North America 17: 227–241. https://doi.org/10.1016/S0891-5520(03)00005-9 [Google Scholar] [CrossRef]
Freeman GJ, Long AJ, Iwai Y, Bourque K, Chernova T et al. (2000). Engagement of the PD-1 immunoinhibitory receptor by a novel B7 family member leads to negative regulation of lymphocyte activation. Journal of Experimental Medicine 192: 1027–1034. https://doi.org/10.1084/jem.192.7.1027 [Google Scholar] [PubMed] [CrossRef]
Gonzalez PS, O’prey J, Cardaci S, Barthet VJ, Sakamaki A et al. (2018). Mannose impairs tumour growth and enhances chemotherapy. Nature 563: 719–723. https://doi.org/10.1038/s41586-018-0729-3 [Google Scholar] [PubMed] [CrossRef]
Hanahan D (2022). Hallmarks of cancer: New dimensions. Cancer Discovery 12: 31–46. https://doi.org/10.1158/2159-8290.CD-21-1059 [Google Scholar] [PubMed] [CrossRef]
Harbeck N, Penault-Llorca F, Cortes J, Gnant M, Houssami N, Poortmans P, Ruddy K, Tsang J, Cardoso F (2019). Breast cancer. Nature Reviews Disease Primers 5: 66. https://doi.org/10.1038/s41572-019-0111-2 [Google Scholar] [PubMed] [CrossRef]
Hatton NE, Baumann CG, Fascione MA (2020). Developments in mannose-based treatments for uropathogenic Escherichia coli-induced urinary tract infections. ChemBioChem 22: 613–629. https://doi.org/10.1002/cbic.202000406 [Google Scholar] [PubMed] [CrossRef]
Herman RH (1971). Mannose metabolism. I. The American Journal of Clinical Nutrition 24: 488–498. https://doi.org/10.1093/ajcn/24.4.488 [Google Scholar] [PubMed] [CrossRef]
Hu X, Shi Y, Zhang P, Miao M, Zhang T, Jiang B (2016). D-mannose: Properties, production, and applications: An overview. Comprehensive Reviews in Food Science and Food Safety 15: 773–785. https://doi.org/10.1111/1541-4337.12211 [Google Scholar] [PubMed] [CrossRef]
Hu C, Xuan Y, Zhang X, Liu Y, Yang S, Yang K (2022). Immune cell metabolism and metabolic reprogramming. Molecular Biology Reports 49: 9783–9795. https://doi.org/10.1007/s11033-022-07474-2 [Google Scholar] [PubMed] [CrossRef]
Jiang Q, Wei B, You M, Zhou X (2023). D-mannose blocks the interaction between keratinocytes and Th17 cells to alleviate psoriasis by inhibiting HIF-1α/CCL20 in mice. International Immunopharmacology 118: 110087. https://doi.org/10.1016/j.intimp.2023.110087 [Google Scholar] [PubMed] [CrossRef]
Jokela TA, Kuokkanen J, Karna R, Pasonen-Seppanen S, Rilla K, Kossi J, Laato M, Tammi MI (2013). Mannose reduces hyaluronan and leukocytes in wound granulation tissue and inhibits migration and hyaluronan-dependent monocyte binding. Wound Repair and Regeneration 21: 247–255. https://doi.org/10.1111/wrr.12022 [Google Scholar] [PubMed] [CrossRef]
Jung J, Zeng H, Horng T (2019). Metabolism as a guiding force for immunity. Nature Cell Biology 21: 85–93. https://doi.org/10.1038/s41556-018-0217-x [Google Scholar] [CrossRef]
Konesan J, Liu L, Mansfield KJ (2022). The clinical trial outcomes of cranberry, D-mannose and NSAIDs in the prevention or management of uncomplicated urinary tract infections in women: A systematic review. Pathogens 11: 1471. https://doi.org/10.3390/pathogens11121471 [Google Scholar] [PubMed] [CrossRef]
Koppenol WH, Bounds PL, Dang CV (2011). Otto Warburg’s contributions to current concepts of cancer metabolism. Nature Reviews Cancer 11: 325–337. https://doi.org/10.1038/nrc3038 [Google Scholar] [PubMed] [CrossRef]
Kovacs SB, Miao EA (2017). Gasdermins: Effectors of pyroptosis. Trends in Cell Biology 27: 673–684. https://doi.org/10.1016/j.tcb.2017.05.005 [Google Scholar] [PubMed] [CrossRef]
Kranjčec B, Papeš D, Altarac S (2013). D-mannose powder for prophylaxis of recurrent urinary tract infections in women: A randomized clinical trial. World Journal of Urology 32: 79–84. https://doi.org/10.1007/s00345-013-1091-6 [Google Scholar] [PubMed] [CrossRef]
Krawczyk CM, Holowka T, Sun J, Blagih J, Amiel E et al. (2010). Toll-like receptor-induced changes in glycolytic metabolism regulate dendritic cell activation. Blood 115: 4742–4749. https://doi.org/10.1182/blood-2009-10-249540 [Google Scholar] [PubMed] [CrossRef]
Krogfelt KA, Bergmans H, Klemm P (1990). Direct evidence that the FimH protein is the mannose-specific adhesin of Escherichia coli type 1 fimbriae. Infection and Immunity 58: 1995–1998. https://doi.org/10.1128/iai.58.6.1995-1998.1990 [Google Scholar] [PubMed] [CrossRef]
Kyriakides R, Jones P, Somani BK (2021). Role of D-mannose in the prevention of recurrent urinary tract infections: Evidence from a systematic review of the literature. European Urology Focus 7: 1166–1169. https://doi.org/10.1016/j.euf.2020.09.004 [Google Scholar] [PubMed] [CrossRef]
Lenger SM, Bradley MS, Thomas DA, Bertolet MH, Lowder JL, Sutcliffe S (2020). D-mannose vs other agents for recurrent urinary tract infection prevention in adult women: A systematic review and meta-analysis. American Journal of Obstetrics and Gynecology 223: 265.e1–265.e13. https://doi.org/10.1016/j.ajog.2020.05.048 [Google Scholar] [PubMed] [CrossRef]
Lenger SM, Chu CM, Ghetti C, Durkin MJ, Jennings Z, Wan F, Sutcliffe S, Lowder JL (2023). D-mannose for recurrent urinary tract infection prevention in postmenopausal women using vaginal estrogen: A randomized controlled trial. Urogynecology 29: 367–377. https://doi.org/10.1097/SPV.0000000000001270 [Google Scholar] [PubMed] [CrossRef]
Lieu EL, Kelekar N, Bhalla P, Kim J (2021). Fructose and mannose in inborn errors of metabolism and cancer. Metabolites 11: 479. https://doi.org/10.3390/metabo11080479 [Google Scholar] [PubMed] [CrossRef]
Lin Z, Miao J, Zhang T, He M, Zhou X, Zhang H, Gao Y, Bai LH (2021). D-mannose suppresses osteoarthritis development in vivo and delays IL-1β-induced degeneration in vitro by enhancing autophagy activated via the AMPK pathway. Biomedicine & Pharmacotherapy 135: 111199. https://doi.org/10.1016/j.biopha.2020.111199 [Google Scholar] [PubMed] [CrossRef]
Luo J, Li Y, Zhai Y, Liu Y, Zeng J et al. (2022). D-mannose ameliorates DNCB-induced atopic dermatitis in mice and TNF-α-induced inflammation in human keratinocytes via mTOR/NF-κB pathway. International Immunopharmacology 113: 109378. https://doi.org/10.1016/j.intimp.2022.109378 [Google Scholar] [PubMed] [CrossRef]
Lv L, Xu Z, Zhao M, Gao J, Jiang R, Wang Q, Shi XY (2022). Mannose inhibits Plasmodium parasite growth and cerebral malaria development via regulation of host immune responses. Frontiers in Immunology 13: 859228. https://doi.org/10.3389/fimmu.2022.859228 [Google Scholar] [PubMed] [CrossRef]
Maloy KJ, Powrie F (2011). Intestinal homeostasis and its breakdown in inflammatory bowel disease. Nature 474: 298–306. https://doi.org/10.1038/nature10208 [Google Scholar] [PubMed] [CrossRef]
Mueckler M, Thorens B (2013). The SLC2 (GLUT) family of membrane transporters. Molecular Aspects of Medicine 34: 121–138. https://doi.org/10.1016/j.mam.2012.07.001 [Google Scholar] [PubMed] [CrossRef]
Olenchock BA, Rathmell JC, Heiden MGV (2017). Biochemical underpinnings of immune cell metabolic phenotypes. Immunity 46: 703–713. https://doi.org/10.1016/j.immuni.2017.04.013 [Google Scholar] [PubMed] [CrossRef]
O’Neill LA, Kishton RJ, Rathmell J (2016). A guide to immunometabolism for immunologists. Nature Reviews Immunology 16: 553–565. https://doi.org/10.1038/nri.2016.70 [Google Scholar] [PubMed] [CrossRef]
Pavlova NN, Thompson CB (2016). The emerging hallmarks of cancer metabolism. Cell Metabolism 23: 27–47. https://doi.org/10.1016/j.cmet.2015.12.006 [Google Scholar] [PubMed] [CrossRef]
Pearce EJ, Everts B (2015). Dendritic cell metabolism. Nature Reviews Immunology 15: 18–29. https://doi.org/10.1038/nri3771 [Google Scholar] [PubMed] [CrossRef]
Pearce EL, Pearce EJ (2013). Metabolic pathways in immune cell activation and quiescence. Immunity 38: 633–643. https://doi.org/10.1016/j.immuni.2013.04.005 [Google Scholar] [PubMed] [CrossRef]
Quattrone C, Manfredi C, Napolitano L, Ferraro A, Distefano C et al. (2023). D-mannose plus saccharomyces boulardii to prevent urinary tract infections and discomfort after cystoscopy: A single-center prospective randomized pilot study. Medicina-Lithuania 59: 1165. https://doi.org/10.3390/medicina59061165 [Google Scholar] [PubMed] [CrossRef]
Radulescu D, David C, Turcu FL, Spataru DM, Popescu P, Vacaroiu IA (2020). Combination of cranberry extract and D-mannose—possible enhancer of uropathogen sensitivity to antibiotics in acute therapy of urinary tract infections: Results of a pilot study. Experimental and Therapeutic Medicine 20: 3399–3406. https://doi.org/10.3892/etm.2020.8970 [Google Scholar] [PubMed] [CrossRef]
Ramos GP, Papadakis KA (2019). Mechanisms of disease: Inflammatory bowel diseases. Mayo Clinic Proceedings 94: 155–165. https://doi.org/10.1016/j.mayocp.2018.09.013 [Google Scholar] [PubMed] [CrossRef]
Rogers PR, Croft M (1999). Peptide dose, affinity, and time of differentiation can contribute to the Th1/Th2 cytokine balance. The Journal of Immunology 163: 1205–1213. https://doi.org/10.4049/jimmunol.163.3.1205 [Google Scholar] [CrossRef]
Rolfsson O, Johannsson F, Magnusdottir M, Paglia G, Sigurjonsson OE, Bordbar A, Palsson S, Brynjólfsson S, Guðmundsson S, Palsson B (2017). Mannose and fructose metabolism in red blood cells during cold storage in SAGM. Transfusion 57: 2665–2676. https://doi.org/10.1111/trf.14266 [Google Scholar] [PubMed] [CrossRef]
Saito Y, Kinoshita M, Yamada A, Kawano S, Liu HS et al. (2021). Mannose and phosphomannose isomerase regulate energy metabolism under glucose starvation in leukemia. Cancer Science 112: 4944–4956. https://doi.org/10.1111/cas.15138 [Google Scholar] [PubMed] [CrossRef]
Schuette V, Embgenbroich M, Ulas T, Welz M, Schulte-Schrepping J et al. (2016). Mannose receptor induces T-cell tolerance via inhibition of CD45 and up-regulation of CTLA-4. Proceedings of the National Academy of Sciences 113: 10649–10654. https://doi.org/10.1073/pnas.1605885113 [Google Scholar] [PubMed] [CrossRef]
Sharma V, Freeze HH (2011). Mannose efflux from the cells: A potential source of mannose in blood. Journal of Biological Chemistry 286: 10193–10200. https://doi.org/10.1074/jbc.M110.194241 [Google Scholar] [PubMed] [CrossRef]
Sharma V, Ichikawa M, Freeze HH (2014). Mannose metabolism: More than meets the eye. Biochemical and Biophysical Research Communications 453: 220–228. https://doi.org/10.1016/j.bbrc.2014.06.021 [Google Scholar] [PubMed] [CrossRef]
Sharma V, Smolin J, Nayak J, Ayala JE, Scott DA, Peterson SN, Freeze HH (2018). Mannose alters gut microbiome, prevents diet-induced obesity, and improves host metabolism. Cell Reports 24: 3087–3098. https://doi.org/10.1016/j.celrep.2018.08.064 [Google Scholar] [PubMed] [CrossRef]
Shen X, Wang H, Weng C, Jiang H, Chen J (2021). Caspase 3/GSDME-dependent pyroptosis contributes to chemotherapy drug-induced nephrotoxicity. Cell Death & Disease 12: 186. https://doi.org/10.1038/s41419-021-03458-5 [Google Scholar] [PubMed] [CrossRef]
Shi LZ, Wang R, Huang G, Vogel P, Neale G, Green DR, Chi H (2011). HIF1α-dependent glycolytic pathway orchestrates a metabolic checkpoint for the differentiation of TH17 and Treg cells. Journal of Experimental Medicine 208: 1367–1376. https://doi.org/10.1084/jem.20110278 [Google Scholar] [PubMed] [CrossRef]
Steinman RM, Cohn ZA (1973). Identification of a novel cell type in peripheral lymphoid organs of mice. The Journal of Experimental Medicine 137: 1142–1162. https://doi.org/10.1084/jem.137.5.1142 [Google Scholar] [CrossRef]
Tannahill GM, Curtis AM, Adamik J, Palsson-Mcdermott EM, Mcgettrick AF, Goel G, Frezza C, Bernard NJ, Kelly B, Foley NH (2013). Succinate is an inflammatory signal that induces IL-1β through HIF-1α. Nature 496: 238–242. https://doi.org/10.1038/nature11986 [Google Scholar] [PubMed] [CrossRef]
Taylor PR, Martinez-Pomares L, Stacey M, Lin HH, Brown GD, Gordon S (2005). Macrophage receptors and immune recognition. Annual Review of Immunology 23: 901–944. https://doi.org/10.1146/annurev.immunol.23.021704.115816 [Google Scholar] [PubMed] [CrossRef]
Torretta S, Scagliola A, Ricci L, Mainini F, di Marco S, Cuccovillo I, Kajaste-Rudnitski A, Sumpton D, Ryan KM, Cardaci S (2020). D-mannose suppresses macrophage IL-1β production. Nature Communications 11: 6343. https://doi.org/10.1038/s41467-020-20164-6 [Google Scholar] [PubMed] [CrossRef]
Tu XY, Qin B, Zhang Y, Zhang C, Kahila M et al. (2019). PD-L1 (B7-H1) competes with the RNA exosome to regulate the DNA damage response and can be targeted to sensitize to radiation or chemotherapy. Molecular Cell 74: 1215–1226. https://doi.org/10.1016/j.molcel.2019.04.005 [Google Scholar] [PubMed] [CrossRef]
van der Zande HJP, Nitsche D, Schlautmann L, Guigas B, Burgdorf S (2021). The mannose receptor: From endocytic receptor and biomarker to regulator of (Meta)Inflammation. Frontiers in Immunology 12: 765034. https://doi.org/10.3389/fimmu.2021.765034 [Google Scholar] [PubMed] [CrossRef]
Vander Heiden MG, Cantley LC, Thompson CB (2009). Understanding the Warburg effect: The metabolic requirements of cell proliferation. Science 324: 1029–1033. https://doi.org/10.1126/science.1160809 [Google Scholar] [PubMed] [CrossRef]
Wang YP, Gao WQ, Shi XY, Ding JJ, Liu W, He HB, Wang K, Shao F (2017). Chemotherapy drugs induce pyroptosis through caspase-3 cleavage of a gasdermin. Nature 547: 99–103. https://doi.org/10.1038/nature22393 [Google Scholar] [PubMed] [CrossRef]
Wang H, Teng X, Abboud G, Li W, Ye S, Morel L (2021). D-mannose ameliorates autoimmune phenotypes in mouse models of lupus. BMC Immunology 22: 1–12. https://doi.org/10.1186/s12865-020-00392-7 [Google Scholar] [PubMed] [CrossRef]
Warburg O (1956). On respiratory impairment in cancer cells. Science 124: 269–270. https://doi.org/10.1126/science.124.3215.269 [Google Scholar] [CrossRef]
Wik JA, Skalhegg BS (2022). T cell metabolism in infection. Frontiers in Immunology 13: 840610. https://doi.org/10.3389/fimmu.2022.840610 [Google Scholar] [PubMed] [CrossRef]
Woodley K, Dillingh LS, Giotopoulos G, Madrigal P, Rattigan KM et al. (2023). Mannose metabolism inhibition sensitizes acute myeloid leukaemia cells to therapy by driving ferroptotic cell death. Nature Communications 14: 2132. https://doi.org/10.1038/s41467-023-37652-0 [Google Scholar] [PubMed] [CrossRef]
Worthington JJ, Kelly A, Smedley C, Bauche D, Campbell S, Marie JC, Travis MA (2015). Integrin αvβ8-mediated TGF-β activation by effector regulatory T cells is essential for suppression of T-cell-mediated inflammation. Immunity 42: 903–915. https://doi.org/10.1016/j.immuni.2015.04.012 [Google Scholar] [PubMed] [CrossRef]
Wu H, Zhang W, Mu W (2019). Recent studies on the biological production of D-mannose. Applied Microbiology and Biotechnology 103: 8753–8761. https://doi.org/10.1007/s00253-019-10151-3 [Google Scholar] [PubMed] [CrossRef]
Xia W, Li Y, Wu M, Jin Q, Wang Q, Li SH, Huang SM, Zhang AH, Zhang Y, Jia ZJ (2021). Gasdermin E deficiency attenuates acute kidney injury by inhibiting pyroptosis and inflammation. Cell Death & Disease 12: 139. https://doi.org/10.1038/s41419-021-03431-2 [Google Scholar] [PubMed] [CrossRef]
Xiao P, Hu Z, Lang J, Pan T, Mertens RT et al. (2023a). Mannose metabolism normalizes gut homeostasis by blocking the TNF-α-mediated proinflammatory circuit. Cellular & Molecular Immunology 20: 119–130. https://doi.org/10.1038/s41423-022-00955-1 [Google Scholar] [PubMed] [CrossRef]
Xiao Y, Yu TJ, Xu Y, Ding R, Wang YP, Jiang YZ, Shao ZM (2023b). Emerging therapies in cancer metabolism. Cell Metabolism 35: 1283–1303. https://doi.org/10.1016/j.cmet.2023.07.006 [Google Scholar] [PubMed] [CrossRef]
Yin L, Duan JJ, Bian XW, Yu SC (2020). Triple-negative breast cancer molecular subtyping and treatment progress. Breast Cancer Research 22: 61. https://doi.org/10.1186/s13058-020-01296-5 [Google Scholar] [PubMed] [CrossRef]
Zhang DF, Chia C, Xue J, Jin WW, Kasagi S et al. (2017). D-mannose induces regulatory T cells and suppresses immunopathology. Nature Medicine 23: 1036–1045. https://doi.org/10.1038/nm.4375 [Google Scholar] [PubMed] [CrossRef]
Zhang R, Yang Y, Dong W, Lin M, He J et al. (2022). D-mannose facilitates immunotherapy and radiotherapy of triple-negative breast cancer via degradation of PD-L1. Proceedings of the National Academy of Sciences 119: e2114851119. https://doi.org/10.1073/pnas.2114851119 [Google Scholar] [PubMed] [CrossRef]
Zhong WD, Zhu JG, Deng YL, Liu R, Cai ZD, Han ZD, Feng YF, Cai SH, Chen QB (2022). Mannose inhibits the growth of prostate cancer through a mitochondrial mechanism. Asian Journal of Andrology 24: 540–548. https://doi.org/10.4103/aja2021104 [Google Scholar] [PubMed] [CrossRef]
Zhou XM, Zheng YC, Sun WT, Zhang ZZ, Liu JQ, Yang WK, Yuan WX, Yi YT, Wang J, Liu J (2021). D-mannose alleviates osteoarthritis progression by inhibiting chondrocyte ferroptosis in a HIF-2 α-dependent manner. Cell Proliferation 54: e13134. https://doi.org/10.1111/cpr.13134 [Google Scholar] [PubMed] [CrossRef]
Zygmunt BM, Wegrzyn A, Gajska W, Yevsa T, Chodaczek G, Guzman CA (2018). Mannose metabolism is essential for Th1 cell differentiation and IFN-γ production. The Journal of Immunology 201: 1400–1411. https://doi.org/10.4049/jimmunol.1700042 [Google Scholar] [PubMed] [CrossRef]
Cite This Article
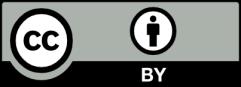
This work is licensed under a Creative Commons Attribution 4.0 International License , which permits unrestricted use, distribution, and reproduction in any medium, provided the original work is properly cited.