Open Access
ARTICLE
Effects of arbuscular mycorrhizal fungi and plant growth-promoting rhizobacteria on growth and reactive oxygen metabolism of tomato fruits under low saline conditions
Anhui Provincial Key Laboratory of the Conservation and Exploitation of Biological Resources, College of Life Sciences, Anhui Normal University, Wuhu, 241000, China
* Corresponding Author: XIANCAN ZHU. Email:
(This article belongs to the Special Issue: Mycorrhizal Fungal Roles in Stress Tolerance of Plants)
BIOCELL 2022, 46(12), 2575-2582. https://doi.org/10.32604/biocell.2022.021910
Received 16 February 2022; Accepted 18 April 2022; Issue published 10 August 2022
Abstract
Land salinization is a major form of land degradation, which is not conducive to the growth and quality of fruits and vegetables. Plant salt tolerance can be enhanced by arbuscular mycorrhizal fungi (AMF) or plant growth-promoting rhizobacteria (PGPR). This study examined the effects of inoculation with PGPR singly or in combination with AMF, on the growth and quality of tomato fruits under low saline conditions. Tomatoes were cultivated in a greenhouse with sterilized soil, inoculated with PGPR, AMF, or co-inoculated with PGPR and AMF, and NaCl solution (1%) was added to the soil. The results indicated that AMF + PGPR decreased the roots and shoot biomass accumulation, and increased the number and fresh biomass in tomato fruits to a certain extent compared with non-inoculated plants. PGPR and AMF mediated the level of reactive oxygen and lipid peroxidation, the accumulation of antioxidants, and the activity of antioxidant enzymes, including proanthocyanidins, flavonoids, ascorbic acid, superoxide dismutase, peroxidase, and total antioxidant capacity. Furthermore, PGPR, AMF, and PGPR + AMF improved the overall osmotic adjustments and accumulation of soluble sugars and soluble proteins. Therefore, the AMF-Funneliformis mosseae and PGPR-Bacillus subtilis can potentially alleviate the adverse effects of salt stress and be applied as a biofertilizer in agricultural practice.Keywords
Abbreviations
AMF: | arbuscular mycorrhizal fungi |
PGPR: | plant growth-promoting rhizobacteria |
H2O2: | hydrogen peroxide |
MDA: | malondialdehyde |
SOD: | superoxide dismutase |
POD: | peroxidase |
T-AOC: | total antioxidant capacity |
TSS: | total soluble sugar |
TSP: | total soluble protein |
In the past decades, continuous deterioration of land salinization has led to a sharp decline in arable land worldwide (Gong et al., 2020). It is estimated that salinization will threaten 50% of arable land in the next 30 years (Chandrasekaran et al., 2014). In recent years, extensive utilization of chemical fertilizer has led to increasing land salinization and agricultural pollution (Mokhtar et al., 2020), including vegetables such as tomatoes (Solanum lycopersicum). Tomato is one of the most nutritious fruits and vegetables consumed worldwide; it is an excellent source of vitamin C, sugar, and natural antioxidants (Chohan and Perveen, 2015). However, salt stress has a deleterious effect on tomato growth, which represses seed germination, reduces the survival rate, and decreases nutrient biomass (Egamberdieva et al., 2019). Several studies have demonstrated colonization of microorganisms inoculated from symbiotic associations with host-plant, which is an effective strategy to alleviate the detrimental effects of salt stress (Kumar et al., 2014; Mukhopadhyay et al., 2021).
Arbuscular mycorrhizal fungi (AMF) are ubiquitous in different soil types, and can infect the roots of most plants including tomatoes, forming the AM structure. Then, a beneficial symbiotic association is established between AMF and the hos-plant (Shi et al., 2021; Yang et al., 2021). In this association, AMF colonization improves the roots of host-plant growth, promoting the uptake of nutrients, including nitrogen, phosphorus, potassium, and so on (Baum et al., 2015). Under salt stress, AMF re-establishes ion homeostasis to remedy insufficient nutrients, enhancing the activity of antioxidant enzymes to stimulate the antioxidant defense system, and improve osmotic adjustment of the host-plant (He et al., 2007; Li et al., 2020; Pan et al., 2020); thus, effectively enhancing the salt tolerance of plants. Meanwhile, AMF upgrades soil fertility and maintains the balance of soil pH by increasing or decreasing the mineral content of the saline soil (Zhang et al., 2011).
Plant growth-promoting rhizobacteria (PGPR) generally include Pseudomonas, Bacillus, Enterobacter, Rhizobia, phosphate solubilizing bacteria, and Erwinia. PGPRs have been shown to promote plant growth and yield under abiotic as well as biotic conditions (Srividhya et al., 2020; Kerbab et al., 2021). Additionally, PGPRs are an important functional component of biofertilizers in laboratory research and agricultural production (Vessey, 2003; Salme et al., 2017). Numerous studies have indicated the promotion mechanisms of PGPR, including increasing the activity of antioxidant enzymes and metabolite accumulation and regulating the content of plant endogenous hormones (Panwar et al., 2016; Qi et al., 2021). Apart from this, most of the phosphorus is present in solid form in the soil, which is difficult to be harnessed by plants; however, previous studies have reported that PGPR improve the plant uptake of mineral elements, especially phosphorus (Dilfuza et al., 2017; Cordero et al., 2018).
This is a significant amount of important information on the physiology and genetics of the tomato, which is effectively cultivated as a model crop in the study of saline land reclamation and utilization of biofertilizers for sustainable agriculture. (Cuartero and Fernández-Muñoz, 1998; Garcia-Gonzalez and Sommerfeld, 2016). However, there has not been much focus on the influence of the inoculation of beneficial microorganisms on tomato fruits in saline soil, especially under low saline conditions. Therefore, this work aimed to (i) compare the effects on fruit growth, antioxidant capacity, and accumulation of soluble substances under different inoculations and (ii) recognize the most effective inoculation treatment of PGPR-Bacillus subtilis and AMF-Funneliformis mosseae under a low saline condition in a pot experiment.
Plant materials and growth conditions
Tomato (cultivar Dongshenghong; provided by the company of Beijing Dongsheng Seed Industry, China) seeds of consistent size were selected, surface sterilized with 75% ethanol solution for 1 min, and rinsed with sterile distilled water three times. Three seeds were sown in separate disinfected pots filled with 300 g autoclaved soil (0.11 MPa, 121°C, 2 h). The pots were randomly placed in a greenhouse, and the temperature was maintained in the region of 25°C. Seedlings were reduced to one seedling per pot 1.5 weeks after emergence.
The inocula of B. subtilis (PGPR; 108 CFU mL–1) and F. mosseae (AMF) were preserved in our lab. The AMF inoculum was a mixture of vermiculite with spores, hyphae, and root residues. For the inoculation treatments, 10 g AMF inoculum (The spore density of 2000 per 10 g inoculum) was mixed well with soil.
The experiment comprised two treatment factors: inoculation (non-inoculation control, AMF, PGPR, and AMF + PGPR treatment) and salinity (control and salt treatments). A completely randomized block design with four replicates was used. For salt treatments, 180 mL (1%) NaCl solution was added to the soil on three consecutive days after three weeks, and sterile distilled water of the same volume was applied as the control. The pots were irrigated regularly with sterile distilled water and 1/2 Hoagland nutrient solution (three times a week) to orient illumination (>500 μmol m−2 s−1 photosynthetic active), temperature (25/16 ± 2°C day/night) and humidity (60%).
After three months, the tomato plants were harvested. First, plant height was measured, and the fruits were picked to determine their number and fresh mass. The shoots and roots were washed with deionized water and dried in a high-temperature oven at 80°C for three days. Then the dry mass of fruits, shoots, and roots was measured.
Fresh fruits were homogenized in 5 mL phosphate buffer (0.1 mol L−1, pH 7.8) and centrifuged at 10,000 × g for 20 min at 4°C, and the supernatant extract was used for assays to estimate hydrogen peroxide (H2O2), malondialdehyde (MDA), proanthocyanidins, flavonoids, ascorbic acid, superoxide dismutase (SOD), peroxidase (POD), total antioxidant capacity (T-AOC), sucrose, fructose, total soluble sugar (TSS) and total soluble protein (TSP) content.
The H2O2 content was determined with the test described by Zhang and Qu (2004). The extract (1 mL) was mixed with 0.1 mL titanium sulphate solution (5%) and 0.2 mL concentrated ammonia. The mixture formed sediment after centrifugation at 3,000 × g for 10 min, and the supernatant was discarded. Sediment was washed with acetone solution until no plant pigment was observed, then 5 mL H2SO4 (2 mol L−1) was added. The mixed solution and washing liquid were transferred to a volumetric flask (10 mL). The absorbance was read by a spectrophotometer (Shimadzu Corporation, Kyoto, Japan; the same as below) at 415 nm to calculate H2O2 content.
The MDA content was determined with the thiobarbituric acid (TBA) test described by Zhang and Qu (2004). One milliliter extract was added to 2 mL 0.6% TBA, which was placed in a boiling water bath for 15 min, then cooled rapidly, and centrifuged to obtain the supernatant. Absorbance was read at 600, 532, and 452 nm. Tissue MDA concentration was calculated according to the formula: MDA (μmol g−1 fw) = (6.45 × (D532 − D600) − 0.56 × D450) × 0.015/W.
The SOD activity was measured according to Bai et al. (1996), based on the inhibition of SOD to reduce nitroblue tetrazolium (NBT) by photochemically generated superoxide radicals. The reaction mixture contained 50 mM phosphate buffer at pH 7.8, 14 mM methionine, 75 µM NBT, 0.1 µM EDTA, 4 µM riboflavin, and the required amount of extract. One unit of SOD was defined as the amount of extract required to inhibit the reduction rate of NBT by 50% at 25°C.
The POD activity was determined by guaiacol oxidation (Bai et al., 1996) in a reaction mixture containing 100 mL phosphate buffer (0.1 mol L−1, pH 6.0), 56 μL guaiacol, and 38 μL H2O2 (30%). Three milliliters reaction solution was added to 1 mL extract. Compared with phosphate buffer (0.1 mol L−1, pH 7.8) as the control group, the absorbance was recorded immediately at 470 nm per 1 min three times. According to variation of values in absorbance and standard curve, POD activity was calculated.
The content of proanthocyanidins, flavonoids, ascorbic acid, T-AOC, sucrose, and fructose was determined according to the instructions provided in the testing kits (Jiancheng Bioengineering Institute, Nanjing, Jiangsu, China).
TSS content was determined by the anthrone method (Zhang and Qu, 2004) using distilled water as the standard. The reaction mixture contained 1 mL extract and 5 mL anthrone agent (100 mg anthrone + 100 mL 76% H2SO4). This was placed in a boiling water bath for 10 min, cooled, and the absorbance was read at 620 nm. The soluble sugar content was calculated according to the formula: C (μg g−1) = A × N/W.
TSP content was determined using the Coomassie brilliant blue G-250 method (Zhang and Qu, 2004). The reaction mixture contained 0.1 mL extract and 5 mL G-250 protein solution agent (100 mg G-250 + 50 mL 95% ethanol + 100 mL 85% orthophosphoric acid + sufficient distilled water; 1000 mL in total). The absorbance was read at 595 nm. The soluble protein content was calculated according to the formula: C (μg) = C × V/W × a.
The experimental data were assessed with correlation analysis and one-factor ANOVA using SPSS (version 22.0 for windows). The significance of differences among means was tested using Duncan’s test at a 5% level.
The treatments did not have any significant effect on the height of tomato plants (Fig. 1a). Under non-salt stress condition, the dry mass of shoot and root of single inoculation with PGPR or AMF was higher than that of the control; nevertheless, the opposite was observed under salt stress condition, and the control treatment produced the highest shoot and root dry mass (Figs. 1b and 1c). Although their fruit dry mass was higher than those in the inoculation treatments, only one fruit was observed in a sample plant of non- and salt stress control treatments (Fig. 1d). Single inoculation with PGPR and co-inoculation of PGPR and AMF produced a higher fruit number (by 19%, 39%, respectively) and fresh mass (by 30%, 25%, respectively) (Figs. 1e and 1f). Instead, fruit number and fresh mass in AMF inoculated samples were the lowest among all treatments; meanwhile, those of the control was higher than single inoculation under non-salt stress condition.
Figure 1: Effects of non-inoculation (CK) and inoculation with the PGPR-Bacillus subtilis (BS), AMF- Funneliformis mosseae (GM), and co-inoculation (BS+GM) on plant height (a), shoot (b), root (c) and fruit (d) dry mass, fruit number (e), and fruit fresh mass (f) under non- (NS) and salt stress (S) conditions. Different letters indicate significant differences (P < 0.05) among treatments by Duncan’s test; n = 4.
Reactive oxygen and lipid peroxidation
Under the salt stress condition, fruit H2O2 content was lower in samples inoculated with PGPR (by 13%) treatments than in the control (CK, Fig. 2a). Salt stress increased H2O2 content except in PGPR inoculated samples by 21%, 83%, and 44%, respectively, in CK, BS, GM and BS+GM. Compared with the control, single inoculation and co-inoculation of PGPR and AMF reduced fruit MDA content under non-stress condition by 15%, 19%, and 39%, respectively (Fig. 2b). Under salt-stress, the MDA content of only PGPR inoculated (by 8%) and co-inoculated (by 12.5%) samples were lower than in the control.
Figure 2: Effects of non-inoculation (CK) and inoculation with the PGPR-Bacillus subtilis (BS), AMF- Funneliformis mosseae (GM), and co-inoculation (BS+GM) on fruit H2O2 (a) and MDA content (b) under non- (NS) and salt stress (S) conditions. Different letters indicate significant differences (P < 0.05) among treatments by Duncan’s test; n = 4.
Inoculation with AMF produced the highest fruit proanthocyanidin and flavonoid content under salt-stress but the lowest ascorbic acid content (Figs. 3a–3c). Interestingly, completely opposite results were observed in fruit ascorbic acid and flavonoid content after PGPR inoculation; those in co-inoculated samples were lower than the non- and salt stress control except for ascorbic acid content.
Figure 3: Effects of non-inoculation (CK) and inoculation with the PGPR-Bacillus subtilis (BS), AMF-Funneliformis mosseae (GM), and co-inoculation (BS+GM) on fruit proanthocyanidin (a), flavonoids (b), ascorbic acid content (c), SOD (d) and POD (e) activities, and total antioxidant capacity (f) under non- (NS) and salt stress (S) conditions. Different letters indicate significant differences (P < 0.05) among treatments by Duncan’s test; n = 4.
Under salt stress, SOD activity in the fruit was enhanced (Fig. 3d). Compared with the control, AMF inoculation markedly increased SOD and POD activity by 22% and 61.5%, respectively, under salt-stress conditions. Under the non-salt stress condition, inoculation treatments enhanced SOD activity more than that in the control. However, under the salt-stress condition, POD activity of single- and co-inoculation with PGPR was lower than the control; meanwhile, SOD activity was similar (Fig. 3e). Under salt stress, the enzyme activity of inoculation treatments was in the order of AMF treatment > co-inoculation treatment > PGPR treatment.
Under salt stress conditions, the fruit total antioxidant capacity of PGPR inoculated samples increased significantly compared with that of the control fruits (P < 0.05). Additionally, when compared with salt stress control, AMF and co-inoculation increased fruit total antioxidant capacity as well, by 154% and 110%, respectively, although the difference was not significant (Fig. 3f).
Under salt stress, only inoculation with AMF obviously increased sucrose and fructose content by 19% and 12.4% compared with those in the control, respectively (Figs. 4a and 4b). However, PGPR and PGPR+AMF treatments showed a modest increase (by 3.5% and 5.2%, respectively) in sucrose content and a decrease in fructose content. Fruit total soluble sugar increased (in CK, BS, GM, BS+GM) in response to salt stress by 16%, 66.4%, 35.7%, and 39.5%, respectively, and a similar improvement in total soluble protein was observed except in PGPR treatment (Fig. 4c). Under salt stress, inoculation with AMF significantly increased soluble protein content compared with that of the control (P < 0.05) (Fig. 4d). Besides, soluble protein content in inoculated samples was higher than that in the control under non-salt stress condition while inoculation with only AMF increased soluble sugar content. Generally, inoculation treatments produced higher total content of soluble sugar or protein than those in the control under both non-salt and salt stress conditions.
Figure 4: Effects of non-inoculation (CK) and inoculation with the PGPR-Bacillus subtilis (BS), AMF- Funneliformis mosseae (GM), and co-inoculation (BS+GM) on fruit sucrose content (a), fructose content (b), total soluble sugar content (c) and total soluble protein content (d) under non-salt (NS) and salt stress (S) conditions. Different letters indicate significant differences (P < 0.05) among treatments by Duncan’s test; n = 4.
Salt stress is one of the most significant abiotic stresses that affect the growth and productivity of crops and reduces the arable land. Utilization of PGPR and AMF may alleviate adverse effects of salt stress in many plant species and more positive effects than adverse effects were observed with inoculation treatments in these studies (Hajiboland et al., 2010; Upadhyay et al., 2012; Srividhya et al., 2020). Our results also showed some negative effects under the low saline condition on the growth and reactive oxygen metabolism of tomatoes. Single inoculation and co-inoculation with PGPR and AMF have their advantages. This may be a result of resource distribution in symbionts, in which both microorganisms and host plants gain water and nutrients from the experimental environment.
Under stress conditions, the plant must adjust its morphological variables to propagate in adverse environments (Pan et al., 2020). Our study showed that single inoculation with PGPR or AMF produced a higher shoot and root dry mass than those in the control with no salt stress, which was consistent with Xun et al. (2015) who reported that single inoculation with PGPR or AMF improved the growth of oat plant in saline-alkali soil. However, in our study, contrary results were observed under salt stress, and the improvement in plant height was not obvious between non- and inoculation treatments. This may be due to the concentration of NaCl, which finally inhibits microbial function.
Fruit number, fresh mass, and dry mass are considered important standards to measure the yield of tomatoes (Luitel et al., 2012). Although co-inoculation led to the lowest shoot and root dry mass among treatments, the fruit number and fresh mass of single inoculation with PGPR and especially co-inoculation treatment were higher than those in the control under salt stress. This may be due to the relationship between nourishment and reproductive growth, and also implies the ability of inoculation to increase tomato production. However, under AMF inoculation treatment, shoot and root dry mass, and the fruit number were lower than in the control under both non- and salt stress conditions, possibly because of resource deficiency as reported by Adesemoye et al. (2009), who suggested that insufficient fertilizer content limited AMF to promote the growth of tomato. In the process of tomato cultivation, “single fruit” (only one fruit in a plant) was observed only in non-inoculation treatments, not in inoculation treatments. This may be due to the effects of PGPR and AMF on the number of flower buds, flowers and pollen fertility, and it would be interesting to study this further.
H2O2 is a toxic reactive oxygen species. Excessive H2O2 reduces cellular membrane permeability and adversely affects metabolic pathways (Hashem et al., 2018). As shown in this study, salt stress increased H2O2 content, which finally damaged the cell membrane system. However, H2O2 content was no significant decline except for single inoculation with PGPR. This may be due to the decline in other components of ROS, such as superoxide ions and hydroxyl and peroxide radicals. MDA is an end product of lipid peroxidation and reflects the degree of damage due to stress (Zhu et al., 2010). PGPR and AMF were found to alleviate adverse effects by reducing MDA content when subjected to salt stress (Xun et al., 2015; Hashem et al., 2018). However, our results were not completely concurrent with these, as we observed that single inoculation and co-inoculation decreased MDA content in the absence of salt stress, while the content was higher than in the control after AMF under salt stress. Similar results were observed in the previous study wherein AMF and rhizobacteria affected the physiology and performance of Sulla coronaria plants under salt stress (Hidri et al., 2019); this may be due to the specific type and combination of PGPR and AMF.
The antioxidant defense system can be enhanced to alleviate salt stress (He et al., 2007). Proanthocyanidin, flavonoid, and ascorbic acid are important antioxidants that enhance salt stress tolerance by neutralizing oxygen free radicals (Koes et al., 1994; Li et al., 2021; Raiola et al., 2015). In our study, we observed that single inoculation of AMF increased proanthocyanidin and flavonoid content but decreased ascorbic acid content under salt stress. Instead, PGPR inoculation treatment simply increased ascorbic acid content. This may be because of different regulatory mechanisms of non-enzymatic antioxidants in PGPR and AMF inoculations.
Moreover, SOD can dismutate superoxide radicals to H2O2 and oxygen, and POD is involved in converting H2O2 into water and oxygen. Our results showed that inoculation with PGPR singly increased the SOD and POD activities in tomato fruit compared with those in the control only under no-salt stress. This may be due to different fruit setting stages under inoculation conditions, as documented in tomato graft treatment with B. subtilis, higher activity was observed on day 28 after grafting (Padró et al., 2021). We also found that SOD and POD activities were higher in AMF-only inoculation than in the control under both non-salt and salt stress conditions, which was in line with Huang et al. (2010), who suggested induction in SOD and POD activities in AM symbiosis compared to those in non-AM plants. It implies that AMF inoculation could alleviate injuries due to oxidation to finally enhance the salt stress tolerance of tomatoes. The positive effects of co-inoculation treatments were not obvious, which may be due to the type of PGPR. The T-AOC was measured considering the antioxidant defense system, including enzymatic and non-enzymatic systems. As shown in the results, inoculation with PGPR or AMF significantly enhanced the enzymatic and non-enzymatic systems; this was in agreement with previous reports on Cucumis sativus L. (Hashem et al., 2018) and Vigna radiata L. (Panwar et al., 2016).
Under salt stress, the plant undergoes osmotic adjustments by synthesizing a high concentration of soluble sugar and soluble protein, finally increasing intracellular osmotic (Janah et al., 2021). In plant tissues, sucrose and fructose are common soluble sugars, and we found that AMF enriched their content over the control under salt stress. Similar results were observed in the Oryza sativa L. subsp. indica under water deficit condition (Tisarum et al., 2019). But PGPR single and co-inoculation treatments led to low or negative increments; likewise, in the present study, fruit total soluble sugar and total soluble protein content increased under salt stress condition, except for PGPR inoculation treatment. This is probably because of different experimental designs such as different types of inocula, the combinations, and so on. Nevertheless, the results were consistent with the investigation conducted by Fernandez et al. (2012) who observed an increase in total soluble sugar content under cold stress. This may be due to plant self-rescue mechanisms triggered by adverse environments, and AMF markedly enhanced the total soluble sugar and protein content than in the control. This agrees with the previous study on Poncirus trifoliata in low-zinc soil (Chen et al., 2017). We also found that inoculations improved the total TSS and TSP content than the non-inoculation tomato under non-salt and salt stress conditions, respectively. It implies that single inoculation and co-inoculation with PGPR and especially AMF can possibly ameliorate tomato fruit quality.
To conclude, in the present study, we observed that single inoculation and co-inoculation with PGPR and AMF do not exert exactly the same effects on tomato growth and fruit quality. Inoculation with AMF led to better fruit quality, while PGPR induced higher antioxidant capacity, and co-inoculation gained more yields. Obviously, it is necessary to conduct in-depth studies on more kinds of microbial inoculums under different levels of salt-stress for applications in agriculture production.
Authors’ Contribution: The authors confirm their contribution to the article as follows: WZ conducted the experiment, statistical analysis, and original draft writing. MMZ and KZT designed the idea and helped with manuscript drafting. All activities took place under the guidance and assistance of XCZ. All authors contributed to the experiment and article and finally approved the submitted version.
Availability of Data and Materials: All data generated or analyzed during this study are included in the article.
Ethics Approval: Not applicable.
Funding Statement: This research was funded by the Anhui Province Innovation and Entrepreneurship Training Program for Undergraduates (S2021110370073).
Conflicts of Interest: The authors declare that they have no conflicts of interest to report regarding the present study.
References
Adesemoye AO, Torbert HA, Kloepper JW (2009). Plant growth-promoting rhizobacteria allow reduced application rates of chemical fertilizers. Microbial Ecology 58: 921–929. [Google Scholar]
Bai BZ, Yu SQ, Tian WX, Zhao JY (1996). Plant Physiology. Beijing: China Agricultural Science Press. [Google Scholar]
Baum C, El-Tohamy W, Gruda N (2015). Increasing the productivity and product quality of vegetable crops using arbuscular mycorrhizal fungi: A review. Scientia Horticulturae 187: 131–141. [Google Scholar]
Chandrasekaran M, Boughattas S, Hu SJ, Oh SH, Sa TM (2014). A meta-analysis of arbuscular mycorrhizal effects on plants grown under salt stress. Mycorrhiza 24: 611–625. [Google Scholar]
Chen YY, Hu CY, Xiao JX (2017). Effects of arbuscular mycorrhizal fungi on the growth and zinc uptake of trifoliate orange (Poncirus trifoliata) seedlings grown in low-zinc soil. Journal of Plant Nutrition 40: 324–331. [Google Scholar]
Chohan S, Perveen R (2015). Phytochemical analysis and antifungal efficacy of rhizome extracts of various plants against fusarium wilt and root rot of tomato. International Journal of Agriculture & Biology 17: 1193–1199. [Google Scholar]
Cordero I, Balaguer L, Rincón A (2018). Inoculation of tomato plants with selected PGPR represents a feasible alternative to chemical fertilization under salt stress. Journal of Plant Nutrition and Soil Science 181: 694–703. [Google Scholar]
Cuartero J, Fernández-Muñoz R (1998). Tomato and salinity. Scientia Horticulturae 78: 83–125. [Google Scholar]
Dilfuza E, Stephan W, Dilfuza J, Räsänen Leena A, Hong L (2017). Coordination between Bradyrhizobium and Pseudomonas alleviates salt stress in soybean through altering root system architecture. Journal of Plant Interactions 12: 100–107. [Google Scholar]
Egamberdieva D, Wirth S, Bellingrath-Kimura SD, Mishra J, Arora NK (2019). Salt-tolerant plant growth promoting rhizobacteria for enhancing crop productivity of saline soils. Frontiers in Microbiology 10: 2791. [Google Scholar]
Fernandez O, Theocharis A, Bordiec S, Feil R, Jacquens L, Clement C, Fontaine F, Barka EA (2012). Burkholderia phytofirmans PsJN acclimates grapevine to cold by modulating carbohydrate metabolism. Molecular Plant-Microbe Interactions 25: 496–504. [Google Scholar]
Garcia-Gonzalez J, Sommerfeld M (2016). Biofertilizer and biostimulant properties of the microalga Acutodesmus dimorphus. Journal of Applied Phycology 28: 1051–1061. [Google Scholar]
Gong Y, Chen LJ, Pan SY, Li XW, Xu MJ et al. (2020). Antifungal potential evaluation and alleviation of salt stress in tomato seedlings by a halotolerant plant growth-promoting actinomycete Streptomyces sp. KLBMP5084. Rhizosphere 16: 100262. [Google Scholar]
Hajiboland R, Aliasgharzadeh N, Laiegh SF, Poschenrieder C (2010). Colonization with arbuscular mycorrhizal fungi improves salinity tolerance of tomato (Solanum lycopersicum L.) plants. Plant and Soil 331: 313–327. [Google Scholar]
Hashem A, Alqarawi AA, Radhakrishnan R, AL-Arjani ABF, Aldehaish HA, Egamberdieva D, Abd_Allah EF (2018). Arbuscular mycorrhizal fungi regulate the oxidative system, hormones and ionic equilibrium to trigger salt stress tolerance in Cucumis sativus L. Saudi Journal of Biological Sciences 25: 1102–1114. [Google Scholar]
He ZQ, He CX, Zhang ZB, Zou ZR, Wang HS (2007). Changes of antioxidative enzymes and cell membrane osmosis in tomato colonized by arbuscular mycorrhizae under NaCl stress. Colloids and Surfaces B: Biointerfaces 59: 128–133. [Google Scholar]
Hidri R, Mahmoud OMB, Farhat N, Cordero, Pueyo JJ et al. (2019). Arbuscular mycorrhizal fungus and rhizobacteria affect the physiology and performance of Sulla coronaria plants subjected to salt stress by mitigation of ionic imbalance. Journal of Plant Nutrition and Soil Science 182: 451–462. [Google Scholar]
Huang Z, He CX, He ZQ, Zou ZR, Zhang ZB (2010). The effects of arbuscular mycorrhizal fungi on reactive oxyradical scavenging system of tomato under salt tolerance. Agricultural Sciences in China 9: 1150–1159. [Google Scholar]
Janah I, Meddich A, Elhasnaoui A, Khayat S, Anli M, Boutasknit A, Aissam S, Loutfi K (2021). Arbuscular mycorrhizal fungi mitigates salt stress toxicity in Stevia rebaudiana Bertoni through the modulation of physiological and biochemical responses. Journal of Soil Science and Plant Nutrition 2021: 1–11. [Google Scholar]
Kerbab S, Silini A, Chenari Bouket A, Cherif-Silini H, Eshelli M, El Houda Rabhi N, Belbahri L (2021). Mitigation of NaCl stress in wheat by rhizosphere engineering using salt habitat adapted PGPR halotolerant bacteria. Applied Science 11: 1034. [Google Scholar]
Koes RE, Quattrocchio F, Mol JNM (1994). The flavonoid biosynthetic pathway in plants: Function and evolution. BioEssays 16: 123–132. [Google Scholar]
Kumar A, Dames JF, Gupta A, Sharma S, Ahmad P (2014). Current developments in arbuscular mycorrhizal fungi research and its role in salinity stress alleviation: A biotechnological perspective. Critical Reviews in Biotechnology 35: 461–474. [Google Scholar]
Li X, Liu J, Chang QX, Zhou ZY, Han RL, Liang ZS (2021). Antioxidant and antidiabetic activity of proanthocyanidins from fagopyrum dibotrys. Molecules 26: 2417. [Google Scholar]
Li Z, Wu N, Meng S, Wu F, Liu T (2020). Arbuscular mycorrhizal fungi (AMF) enhance the tolerance of Euonymus maackii Rupr. at a moderate level of salinity. PLoS One 15: e023149. [Google Scholar]
Luitel BP, Adhikari PB, Yoon CS, Kang WH (2012). Yield and fruit quality of tomato (Lycopersicon esculentum Mill.) cultivars established at different planting bed size and growing substrates. Horticulture Environment and Biotechnology 53: 102–107. [Google Scholar]
Mokhtar M, Baslam M, Ben-Laouane R, Anli M, Boutasknit A, Mitsui T, Wahbi S, Meddich A (2020). Alleviation of detrimental effects of salt stress on date palm (Phoenix dactylifera L.) by the application of arbuscular mycorrhizal fungi and/or compost. Frontiers in Sustainable Food Systems 4: 131. [Google Scholar]
Mukhopadhyay R, Sarkar B, Jat HS, Sharma PC, Bolan NS (2021). Soil salinity under climate change: Challenges for sustainable agriculture and food security. Journal of Environmental Management 280: 111736. [Google Scholar]
Padró MDA, Caboni E, Morin KAS, Mercado MAM, Olalde-Portugal V (2021). Effect of Bacillus subtilis on antioxidant enzyme activities in tomato grafting. PeerJ 9: e10984. [Google Scholar]
Pan J, Huang C, Peng F, Zhang WJ, Luo J, Ma SX, Xue X (2020). Effect of arbuscular mycorrhizal fungi (AMF) and plant growth-promoting bacteria (PGPR) inoculations on Elaeagnus angustifolia L. Saline saline Soilsoil. Applied Sciences 10: 945. [Google Scholar]
Panwar M, Tewari R, Nayyar H (2016). Native halo-tolerant plant growth promoting rhizobacteria Enterococcus and Pantoea sp. improve seed yield of Mungbean (Vigna radiata L.) under soil salinity by reducing sodium uptake and stress injury. Physiology and Molecular Biology of Plants 22: 445–459. [Google Scholar]
Qi RX, Lin W, Gong KX, Han ZY, Ma H, Zhang M, Zhang QN, Gao YM, Li JS, Zhang XY (2021). Bacillus co-inoculation alleviated salt stress in seedlings cucumber. Agronomy 11: 966. [Google Scholar]
Raiola A, Tenore GC, Petito R, Ciampalia R, Ritieni A (2015). Improving of nutraceutical features of many important mediterranean vegetables by inoculation with a new commercial product. Current Pharmaceutical Biotechnology 16: 738–746. [Google Scholar]
Salme T, Lawrence B, Julia M, Anthony M, Anne-Charlotte A (2017). Perspectives and challenges of microbial application for crop improvement. Frontiers in Plant Science 8: 49. [Google Scholar]
Shi J, Zhao B, Zheng S, Zhang X, Wang X et al. (2021). A phosphate starvation response-centered network regulates mycorrhizal symbiosis. Cell 184: 5527–5540.e18. [Google Scholar]
Srividhya S, Kumari N, Surendranath R, Jeyakumar P (2020). Role of rhizobacteria in alleviating salt stress. In: Sharma V, Salwan R, Al-Ani LKT (eds.Molecular Aspects of Plant Beneficial Microbes in Agriculture, pp. 279–294. London: Academic Press. [Google Scholar]
Tisarum R, Theerawitaya C, Samphumphuang T, Phisalaphong M, Singh HP, Cha-um S (2019). Promoting water deficit tolerance and anthocyanin fortification in pigmented rice cultivar (Oryza sativa L. subsp. indica) using arbuscular mycorrhizal fungi inoculation. Physiology and Molecular Biology of Plants 25: 821–835. [Google Scholar]
Upadhyay SK, Singh JS, Saxena AK, Singh DP (2012). Impact of PGPR inoculation on growth and antioxidant status of wheat under saline conditions. Plant Biology 14: 605–611. [Google Scholar]
Vessey JK (2003). Plant growth promoting rhizobacteria as biofertilizers. Plant and Soil 255: 571–586. [Google Scholar]
Xun FF, Xie BM, Liu SS, Guo CH (2015). Effect of plant growth-promoting bacteria (PGPR) and arbuscular mycorrhizal fungi (AMF) inoculation on oats in saline-alkali soil contaminated by petroleum to enhance phytoremediation. Environmental Science and Pollution Research 22: 598–608. [Google Scholar]
Yang W, Li S, Wang X, Liu F, Li X, Zhu X (2021). Soil properties and geography shape arbuscular mycorrhizal fungal communities in black land of China. Applied Soil Ecology 167: 104109. [Google Scholar]
Zhang YF, Wang P, Yang YF, Bi Q, Tian SY, Shi XW (2011). Arbuscular mycorrhizal fungi improve reestablishment of Leymus chinensis in bare saline-alkaline soil: Implication on vegetation restoration of extremely degraded land. Journal of Arid Environments 75: 773–778. [Google Scholar]
Zhang ZL, Qu W (2004). Experimental Guidance of Plant Physiology. Beijing: High Education. [Google Scholar]
Zhu X, Song F, Xu H (2010). Influence of arbuscular mycorrhiza on lipid peroxidation and antioxidant enzyme activity of maize plants under temperature stress. Mycorrhiza 20: 325–332. [Google Scholar]
Cite This Article
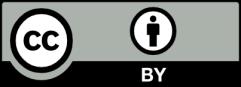
This work is licensed under a Creative Commons Attribution 4.0 International License , which permits unrestricted use, distribution, and reproduction in any medium, provided the original work is properly cited.